新闻动态
Cellular lifespan and senescence signaling in embryonic stem...
2025-07-15
Takumi Miura, Stem Cell Biology Unit andSearch for more papers by this authorMark P. Mattson, Cellular and Molecular Neurosciences Section, Laboratory of Neurosciences, National Institute on Aging, Gerontology Research Center, Baltimore, MD 21224, USA Department of Neuroscience, School of Medicine, Johns Hopkins University, Baltimore, MD 21205, USASearch for more papers by this authorMahendra S. Rao, Corresponding Author Stem Cell Biology Unit and Department of Neuroscience, School of Medicine, Johns Hopkins University, Baltimore, MD 21205, USADr M. S. Rao, Stem Cell Biology Unit, Laboratory of Neurosciences, National Institute on Aging, GRC, 5600 Nathan Shock Drive, Room 4E01, Baltimore, MD 21224, USA. Tel.: +410 558 8204; fax: +410 558 8323; e-mail: raomah@grc.nia.nih.govSearch for more papers by this author Takumi Miura, Stem Cell Biology Unit andSearch for more papers by this authorMark P. Mattson, Cellular and Molecular Neurosciences Section, Laboratory of Neurosciences, National Institute on Aging, Gerontology Research Center, Baltimore, MD 21224, USA Department of Neuroscience, School of Medicine, Johns Hopkins University, Baltimore, MD 21205, USASearch for more papers by this authorMahendra S. Rao, Corresponding Author Stem Cell Biology Unit and Department of Neuroscience, School of Medicine, Johns Hopkins University, Baltimore, MD 21205, USADr M. S. Rao, Stem Cell Biology Unit, Laboratory of Neurosciences, National Institute on Aging, GRC, 5600 Nathan Shock Drive, Room 4E01, Baltimore, MD 21224, USA. Tel.: +410 558 8204; fax: +410 558 8323; e-mail: raomah@grc.nia.nih.govSearch for more papers by this author Give accessShare full text accessShare full-text accessPlease review our Terms and Conditions of Use and check box below to share full-text version of article.I have read and accept the Wiley Online Library Terms and Conditions of UseShareable LinkUse the link below to share a full-text version of this article with your friends and colleagues. Learn more.Copy URLShare a linkShare onEmailFacebookTwitterLinked InRedditWechat Summary Most mammalian cells when placed in culture will undergo a limited number of cell divisions before entering an unresponsive non-proliferating state termed senescence. However, several pathways that are activated singly or in concert can allow cells to bypass senescence at least for limited periods. These include the telomerase pathway required to maintain telomere ends, the p53 and Rb pathways required to direct senescence in response to DNA damage, telomere shortening and mitogenic signals, and the insulin-like growth factor – Akt pathway that may regulate lifespan and cell proliferation. In this review, we summarize recent findings related to these pathways in embryonic stem (ES) cells and suggest that ES cells are immortal because these pathways are tightly regulated. Introduction Most mammalian cells undergo a limited number of cell divisions in culture before entering a state of irreversible proliferative arrest termed replicative senescence (Hayflick & Moorhead, 1961). The number of cell divisions in culture varies with cell type and species and this number has been termed the Hayflick limit. The occurrence of replicative senescence is determined by the number of times that a cell population divides, suggesting that a mitotic clock records cell divisions (Hayflick, 1976). Although senescence appears invariable in most cell types, transformed cells (Smith & Pereira-Smith, 1996), embryonic stem (ES) cells (Thomson etal., 1998) and some somatic cells (Kiyono etal., 1998) appear to be able to bypass senescence. Studies of senescence of somatic cells in culture, and examination of signaling pathways that enable transformed cells to escape senescence, have suggested five major avenues that may operate to extend the Hayflick limit. These pathways, which may be important in human aging and age-associated diseases (Campisi, 2001), include the telomerase pathway, the p53 pathway, the Rb pathway (Lee etal., 2000; Ferbeyre etal., 2002), the insulin-like growth factor (IGF) – Akt kinase pathway (Miyauchi etal., 2004) and the mitochondrial/oxidative stress pathway (reviewed in Szibor & Holtz, 2003). In addition, it has been noted by cell fusion experiments that senescence is dominant over immortality. Microcell fusion experiments show that when immortal cells are fused with non-transformed mortal cells the resulting cells lose their ability to proliferate indefinitely. This strategy has been used successfully to map potential mortality genes and four different loci have been identified. Some genes have been cloned and these include MORFs (mortality factors) and Mortalins (Wadhwa etal., 1994; Bertram etal., 1999). Mutational analysis in C.elegans and yeast has confirmed the importance of many of these pathways and also identified sirtuins, polycomb repressors and proteins involved in DNA repair as being important in regulating prolonged self-renewal. Van Zant and colleagues have taken a forward genetic approach to understand how longevity may be modulated. They have used an elegant breeding strategy to identify loci that have a high probability of containing genes important in stem cell proliferation and longevity (reviewed in Van Zant, 2003). These loci include DNA repair proteins, heat shock proteins and other likely candidates. In most somatic cells, which lack the activity of the telomere-lengthening enzyme telomerase (Greider & Blackburn, 1987; Kim etal., 1994), telomere shortening may contribute to a mitotic clock that records cell divisions (Harley, 1991). p53 and Rb are typically activated during senescence, and enforced expression of either protein induces senescence in some human normal cells (Lee etal., 2000; Ferbeyre etal., 2002). In addition, it has been reported that the activation of the PI3K/Akt pathway increases with cellular senescence and that inhibition of Akt extends the lifespan of primary cultured human endothelial cells (Miyauchi etal., 2004). These studies have highlighted the conserved nature of these pathways but have also suggested that species differences exist. Differences in telomere biology, the role of sirtuins (Langley etal., 2002) and the IGF/Akt pathway have been described. In rodents and humans, many of these studies have been undertaken in immortalized cells or tumor-derived cell lines as no truly immortal somatic cell type (with the exception of ES cells) has been described and it is unclear if some or all of these pathways are required to avoid replicative senescence during normal development. ES cells are a unique population of stem cells that are derived from the inner cell mass of a developing pre-implantation embryo. Unlike most somatic cells, ES cells are spontaneously immortal and appear capable of indefinite self-renewal while retaining their ability to differentiate and contribute to the germ line after blastocyst injection. The ability to self-renew indefinitely is lost as ES cells differentiate to generate somatic tissue. This has suggested to us that ES cell lines may serve as a good model to assess the role of specific pathways that may allow cells to bypass senescence. Gene expression in ES cell lines has been described using a variety of large-scale analytical strategies (Sato etal., 2003; Sperger etal., 2003; Bhattacharya etal., 2004; Brandenberger etal., 2004a; Ginis etal., 2004; Miura etal., 2004) and expression of candidate senescence-related genes has been documented. Comparison of expression of senescence-related genes between ES cells and cells that differentiate from them may highlight key regulatory players. In this review, we focus on four major pathways that regulate cellular senescence and possibly aging. We summarize studies on the telomerase pathway, the p53 pathway, the Rb pathway and the IGF/Akt pathway and correlate these with gene expression results in human ES cells. We suggest that the pattern of gene expression indicates that ES cells are spontaneously immortal because these pathways are active. One mechanism that may underlie the ability of stem cells to replicate indefinitely is the expression of the DNA repair enzyme complex that includes telomerase, an RNA-dependent DNA polymerase that maintains telomere length (Tang etal., 2001; Xu etal., 2001). Analysis of telomerase enzyme levels or activity has shown that high levels are expressed by ES cells (Thomson etal., 1998; Armstrong etal., 2000) and lower levels are expressed in proliferative cells of renewal tissues. In addition, telomerase activity is down-regulated in cells that exit the cell cycle via either terminal differentiation or (reversible) quiescence. Overexpression of the catalytic subunit of telomerase (TERT) allows cells to maintain a normal karyotype and proliferate for substantially prolonged periods beyond the Hayflick limit (reviewed in Forsyth etal., 2002). Reduced telomerase activity leads to a loss of telomeric repeats (TTAGGG) at each successive division due to incomplete replication of telomeric ends (Harley etal., 1990; Blasco etal., 1997). Depending on telomere length (which varies from species to species), after a certain number of divisions, telomeres may be completely lost with subsequent loss of genes at chromosomal ends or abnormal chromosomal replications leading to loss of proliferative potential or cell death (Maser & DePinho, 2002). It is clear that the level of expression of the TERT subunit is important for telomerase activity (Feng etal., 1995; Cong etal., 2002). In contrast to most somatic cells, stem, germ and tumor cells have high telomerase activity through TERT transcriptional up-regulation. One way of regulating telomerase activity appears to be to regulate gene expression, and studies have begun to identify key regulators. The TERT promoter was cloned in 1999 (Cong etal., 1999; Horikawa etal., 1999; Takakura etal., 1999; Greenberg etal., 2004). The core TERT promoter, a region of ∼300bp upstream of the transcriptional start site, lacks a TATA sequence, but contains two E-boxes surrounding several Sp1 binding sites. E-box sites bind several cellular proteins, including the Myc/Mad/Max family of transcription factors (Greenberg etal., 1999; Horikawa etal., 1999; Takakura etal., 1999; Wu etal., 1999; Kyo etal., 2000) (Fig.1). Intriguingly, c-myc is a potent oncogene, up-regulated in many human tumours (Cole & McMahon, 1999). Also, c-myc deficiency can cause a marked reduction in embryo size and a generalized delay in the early development of many organs (Davis & Bradley, 1993; Davis etal., 1993), consistent with a role for c-myc in the self-renewal of embryonic cells. Mechanism of telomerase activation and telomere maintenance. signaling and transcription factors regulating the TERT promoter are indicated. In addition, the figure displays some telomere binding factors that regulate telomere length. Genes in red are up-regulated in human ES cells, and genes shown in green are not detectable by MPSS (see Table1). Orange or blue, respectively, shows transactivators or repressors on TERT promoter. Telomeres are not just simple physical ends but contain telomere specific proteins (TRF1 and 2) that can modulate telomerase activity (Broccoli etal., 1997) and can modulate telomere length and consequently proliferation (van Steensel & de Lange, 1997). Other proteins associated with telomeric DNA influence telomere length regulation (Smogorzewska & De Lange, 2004). A number of proteins that bind telomeric DNA with high specificity and play a role in protection of telomeric ends have been described in Table1. TRF1 and TRF2 form multiprotein complexes that are required for chromosome capping (de Lange, 2002) (Fig.1). These complexes regulate telomerase activity by facilitating its access to telomeric DNA. Additionally, TRF2 is thought to help protect telomeres by restructuring telomeric DNA into T loops in which the 3′ single strand invades the duplex region of the same telomere (van Steensel etal., 1998; Griffith etal., 1999; Munoz-Jordan etal., 2001; Stansel etal., 2001). A telomeric single-strand overhang binding protein has also been identified, termed POT1 (protection of telomeres 1), which regulates telomere elongation (Baumann & Cech, 2001; Baumann etal., 2002; Colgin etal., 2003; Loayza & De Lange, 2003). It is likely that these proteins will be present and regulated in ES cells as they are in transformed cells. The abundance for each listed gene was converted to transcripts per million (Tpm) for the purpose of comparison between human ES cells and EB. Several lines of evidence suggest that the telomerase pathway is active in ES cells and indeed is required for prolonged self-renewal. Human and mouse ES cells have a normal karyotype and maintain high telomerase activity (Kim etal., 1994; Rosler etal., 2004), and human ES cells continue to express high levels of telomerase even after more than 300 population doublings and continuous passage for more than a year in culture (Thomson & Marshall, 1998; Thomson etal., 1998; Rosler etal., 2004). Telomerase activity is down-regulated during differentiation of rodent and human ES cells (T. Miura etal., unpublished observations) as well as in embryonal carcinoma (EC) cells (Kruk etal., 1996). This suppression of telomerase activity in differentiated EC cells requires histone deacetylation in early hTERT gene down-regulation and DNA methylation for maintenance of silencing of the hTERT gene (Lopatina etal., 2003). Both histone deacetylases (HDACs) and methylation are tightly regulated in ES cells (Huntriss etal., 2004; Lee etal., 2004). Expression of TERT is important for ES cell self-renewal in humans and the growth rate of telomerase-deficient ES cells is gradually reduced after more than 300 divisions and is accompanied by continual telomere shortening. Furthermore, telomerase-deficient mouse ES cells cease to grow after 460–480 divisions (Niida etal., 1998) strongly suggesting that telomerase is essential for proliferation in ES cells. In both mouse ES cells as well human ES cells (T. Miura etal., unpublished observations), it appears that myc may also be critical in regulating cell proliferation. Overexpression of c-myc is sufficient to maintain mouse ES cells in an undifferentiated state and blocking myc expression promotes differentiation (S. Dalton etal., unpublished results). However, how alterations in TERT levels influence ES cell differentiation remains to be determined and it remains unclear whether c-Myc activates telomerase directly in ES cells (Armstrong etal., 2000). Two other growth factors are important in up- or down-regulating telomerase activity, basic fibroblast growth factor (bFGF) and transforming growth factor-beta (TGF-β). bFGF is essential for the maintenance of ES cells (Xu etal., 2001). Interestingly, bFGF also up-regulates telomerase activity in human endothelial cells (Kurz etal., 2003). On the other hand, members of the TGF-β superfamily induce differentiation in ES cells (Xu etal., 2002). Moreover, telomerase activity is down-regulated by TGF-β in cancer cells (Katakura etal., 1999, 2003; Lin & Elledge, 2003) (Fig.1). These findings suggest that activation of the bFGF pathway and/or a negative regulation of the TGF-β pathway may be critical for the maintenance of the undifferentiated ES cells. Table1 summarizes levels of expression of TERT and TERT-related proteins as detected by MPSS (Massively Parallel Signature Sequencing) (Brandenberger etal., 2004a; Miura etal., 2004) and validated by EST (expressed sequence tag) scan or microarray (Bhattacharya etal., 2004; Brandenberger etal., 2004b). Most components of the telomerase complex (TERT, TERC, TEP1, hsp90, p23 and dyskerin) (Chang etal., 2002) are present at higher levels in ES cells than in embryoid bodies (EB). TRF1 (ES:EB=421:3) and TRF2 (5:2), which play critical roles to protect and maintain chromosome ends (van Steensel & de Lange, 1997; Smogorzewska etal., 2000), are likewise present at high levels, while antagonists of their activity are up-regulated as ES cells differentiate. In summary, the results suggest that the TERT pathway is active and probably critical for prolonged self-renewal of cells. High telomerase activity is required as in other cell types for maintenance of the ES cell state and these high levels are probably regulated by FGF and TGF-β signaling. Myc is probably important in the TERT pathway and regulates cell cycle gene expression, and TERT interacting/related proteins such as TRF1, POT1 and HSP90 are important modulators. Comparing expression in ES cells and embryoid bodies derived from them suggests that components of the telomeric complex may be critical in maintaining telomeric ends. The protein p53 is a tumor suppressor that normally responds to DNA damage by inducing apoptosis and thereby prevents cell transformation (Oren & Rotter, 1999; Bargonetti & Manfredi, 2002). It is also a critical regulator of the senescence response to various types of cellular signals, including telomere shortenings, DNA damage, oncogene activations and overexpressed tumor suppressor genes (Di Leonardo etal., 1994; Serrano etal., 1997; Lin etal., 1998; Sherr, 1998; Dimri etal., 2000; Pearson etal., 2000) (Fig.2). Besides playing an important role in controlling cellular senescence and proliferation, p53 has also been implicated in regulating cellular differentiation (reviewed in Wolkowicz & Rotter, 1997). In addition, its dominant-negative mutants of p53 inhibit haematopoietic and muscle differentiation, and the forced expression of p53 results in a differentiated phenotype (Soddu etal., 1996). Likewise, several other reports have shown that endogenous p53 levels and activities are modulated upon differentiation. p53 expression is up-regulated during maturation of human haematopoietic cells (Kastan etal., 1991). In addition, p53 has been reported to be down-regulated at the protein and RNA levels during embryogenesis (Louis etal., 1988). These results suggest that p53 is a key integrator of diverse signaling pathways and, by determining whether cells will self-renew, differentiate or die, its regulation plays an important role during early embryonic development. Effects of p53 and pRb pathways on ES cell proliferation. This figure summarizes some of the pathways that are involved in the G1–S transition in differentiated somatic cells. However, it seems that these cell cycle regulatory pathways in undifferentiated ES cells differ significantly from those in differentiated somatic cells. Genes in red are up-regulated in human ES cells, and genes shown in blue are highly detectable in embryoid bodies (EB) by MPSS. Genes shown in green are not detectable by MPSS (see Table2). Orange displays factors or phenotype that might be associated with p53 and Rb pathways. The regulation of p53 does not appear to be critical for ES cells. ES cells, in contrast to all other somatic cells (including somatic stem cells), do not undergo cell-cycle arrest under conditions such as ribonucleotide depletion and DNA damage. Stimuli that would normally activate the p53-mediated cell-cycle arrest pathway in somatic cells appear unable to activate this pathway, despite the fact that mouse ES cells express high levels of p53. It appears that the p53-mediated response is inactive because of cytoplasmic sequestration of p53 and low efficiency of p53 translation to the nucleus (Aladjem etal., 1998; Prost etal., 1998). Moreover, it has been shown that the p53-mediated cell-cycle arrest response is restored upon differentiation of ES cells (Aladjem etal., 1998). Similar results are seen in EC cells. High levels of normal p53 are present in EC cells (Oren etal., 1982) and the expression of p53 is down-regulated upon differentiation of EC cells. Likewise, p53 appears critical only during late stages of normal embryogenesis (Louis etal., 1988; Schmid etal., 1991; MacCallum etal., 1996). Although p53-null mice are viable (Donehower etal., 1992; Jacks etal., 1994), mice lacking mdm2, which is a negative regulator of p53, die at an early embryo stage (Jones etal., 1995; Montes de Oca Luna etal., 1995). Collectively, the data suggest that p53 is important during differentiation and, although it is expressed in ES cells, its activity is inhibited and not required for ES cell self-renewal or early embryogenesis. Consistent with these observations, it has been possible to derive ES cell lines from p53 null animals. We generated the gene expression profile of p53 pathway in the human ES cells and EB by MPSS (Brandenberger etal., 2004a; Miura etal., 2004). Interestingly, p53 was present at very low levels in human ES cells. In addition, levels of INK4a locus gene (p16 and p19) and p21 are low in ES cells and induced as cells differentiate (Table2). These p53-regulated genes have been found in cells undergoing replicative senescence along with p53 and p21CIP proteins (Sherr & DePinho, 2000). Thus, ES cells might not also require p53 for self-renewal and its absence or lack of activity may be important in permitting ES cells to renew indefinitely. The abundance for each listed gene was converted to transcripts per million (Tpm) for the purpose of comparison between human ES cells and EB. How p53 is maintained in an inactive state remains to be determined and may be a promising line of inquiry in defining key regulators of senescence. Mdm2 or a related family member may be important, as may be the ubiquitinylation pathway that regulates p53 activity (Honda etal., 1997; Zhang etal., 2004). The MPSS data suggest that the p53 pathway, which is a critical modulator of senescence, is inactive in human ES cells as well as rodent ES cells, and that this inactivity is correlated with the ability of cells to self-renew indefinitely. Additionally, Donehower and colleagues have recently generated a p53 mutant mouse that appears to display hypermorphic p53 activity. This mutant mouse, the p53+/m mouse, shows reduced longevity and an early onset of phenotypes associated with aging, including organ atrophy and a reduced regenerative response (Tyner etal., 2002), suggesting that stem cell function could be an important component of these phenotypes. Thus, increased p53 activity results in inhibition of stem cell proliferation, apoptosis or differentiation, suggesting that maintaining p53 in an inactive state is required for prolonged self-renewal. Similar to p53, Rb is an important senescence regulator and interacts with p53. Rb and p53 are typically activated during senescence, and enforced expression of either protein induces senescence in some cell types (Lee etal., 2000; Ferbeyre etal., 2002). The Rb/E2F pathway also plays a key role in controlling cell growth by integrating multiple mitogenic and anti-mitogenic stimuli. The components of this pathway are gene families with a high level of structural and functional redundancy and are expressed in an overlapping fashion in most tissues and cell types. Evidence from a variety of studies has suggested that cell cycle activation is co-ordinated by D-type cyclins, which are rate limiting and essential for the progression through the G1 phase of the cell cycle. D-type cyclins bind to and activate the cyclin-dependent kinases Cdk4 and Cdk6, which in turn phosphorylate their downstream target, the retinoblastoma protein Rb (Fig.2). Upon Rb phosphorylation, the E2F transcription factors activate the expression of S-phase genes and thereby induce cell cycle progression. The rise of cyclin D levels in early G1 also serves to titrate p27Kip1/p21Cip1 proteins away from cyclin E/Cdk2 complexes, further accelerating cell cycle progression (reviewed in Coqueret, 2002; Ortega etal., 2002). Intriguingly, Rb and the cell cycle appear to be differently regulated in ES cells as compared with all other somatic cells, raising the possibility that this difference may underlie the ability of ES cells to self-renew indefinitely. Cell division of ES cells is driven by unusually high Cdk2-cyclin A/E activity that is constitutively active throughout the cell cycle (Stead etal., 2002). Hence, cell cycle regulation in ES cells resembles the mode of regulation described for many tumor cell types (Halaban, 1999). These activities account for the abundance of hyperphosphorylated pRb (Savatier etal., 1994) and absence of cell cycle-regulated E2F transcriptional activity (Stead etal., 2002). The current view is that ES cells are deficient in cyclin D-associated Cdk activity (Savatier etal., 1996; Burdon etal., 2002; Jirmanova etal., 2002) and rely on Cdk2 activity to drive the G1–S transition (Stead etal., 2002). This overall scenario indicates that mitogenic signaling is not coupled to the cell cycle machinery and the pRb/E2F pathway is inactive in ES cells (see below and Burdon etal., 2002). As a consequence, ES cells have a very short G1 phase during which hypophosphorylated Rb protein cannot be observed (Savatier etal., 1994). This absence of hypophosphorylated Rb in ES cells may trigger DNA replication right after exit from mitosis and is probably critical for prolonged self-renewal and distinguishes ES cells from all other differentiated cells. Consistent with this hypothesis, which suggests that expression of the Rb family of proteins is low or absent, triple knockout ES cells, in which all three Rb family genes are knocked out, show normal growth rates, and DNA damage does not lead to arrest at G1 as is typical of other cells. Thus, lack of Rb regulation of the cell cycle may be relevant to the high proliferation rate, lack of growth arrest and the absence of senescence of ES cells. The E2F family of transcription factors controls cell proliferation by regulating entry into the cell cycle as well as the G1–S phase transition. E2F proteins bind pocket proteins of the Rb family, consisting of Rb, p107 and p130 (Dyson, 1998). Our MPSS data showed that regulators of D-type cyclins are expressed at relatively higher levels in EB than in ES cells (Table2). Therefore, the p16INK4a/Cyclin D/Rb pathway may also be inactive in human ES cells, consistent with observations in mouse ES cells (Savatier etal., 1996; Burdon etal., 2002; Jirmanova etal., 2002). Altogether, these data support the idea that signaling directed by the pRb pathway is not active in ES cells. Intriguingly, Rb and p53 are regulated differently in mouse and human cells. Ginis etal. (2004) examined the expression of the cell cycle and cell death pathway gene by microarray and showed that whereas the overall regulation was similar, different members of the same family or different pathways were often used to regulate activity. Thus, although both populations show indefinite self-renewal and absence of senescence, the mechanisms by which they achieve this differs. This is reminiscent of the differences in telomerase biology between rodents and humans (reviewed in Artandi & DePinho, 2000; Wright & Shay, 2000; Forsyth etal., 2002). Taken together, these findings strongly suggest that the cell cycle regulatory pathways in undifferentiated ES cells differ significantly from those in differentiated somatic cells and resemble those in transformed cells. This method of regulating the cell cycle may be important in ES cells avoiding senescence as Rb regulation is induced when cells begin to undergo differentiation and lose their ability to bypass senescence. Both mouse and human cells appear similar in their regulation of Rb, although important differences in how Rb is inactivated are observed. Further experiments are required to verify how critical this pathway is in regulating long-term self-renewal, although the current results provide a framework in which to design such experiments. As discussed above, cellular senescence is associated with many molecular mechanisms, including the telomere shortening, p19ARF/p53 and p16INK4a/Rb pathways. An additional key regulator of senescence and organism aging is the IGF/Akt pathway (Fig.3). The importance of this pathway was convincingly demonstrated in mutational analysis in flies and worms (Staveley etal., 1998; Vanfleteren & Braeckman, 1999), and the relative importance of different components of this signaling pathway were established (reviewed in Sulis & Parsons, 2003). Schematic representation of the role of the IGF/Akt pathway in ES cell survival. PTEN dephosphorylates phosphatidylinositol (3,4,5)-trisphosphate (PIP3). A lower level of PIP3 at the membrane decreases the concentration of phosphporylated (active) Akt, thereby down-regulating downstream effectors. Thus, PTEN functions as a negative regulator of Akt. As a result, the IGF/Akt pathway might regulate ES cell survival and cell cycle progression. Genes in red are up-regulated in human ES cells, and genes shown in blue are highly detectable in embryoid bodies (EB) by MPSS. Genes shown in green are not detectable by MPSS (see Table3). Orange displays factors or phenotype that might be associated with the IGF/Akt pathway. Recent genetic analyses have shown that the signaling pathway of insulin/IGF-1/phosphatidylinocitol-3-kinase (PI3K)/Akt (also known as protein kinase B) dramatically extends the lifespan of the nematode C.elegans (Kenyon, 2001; Lin etal., 2001; Longo & Finch, 2003). Likewise, in mammalian cells, activation of Akt has been reported to induce proliferation, enhance cell survival and increase replicative lifespan. Overactivity of this pathway may promote tumorigenesis (Datta etal., 1999; Blume-Jensen & Hunter, 2001; Testa & Bellacosa, 2001). Indeed, overexpression of Akt can transform NIH3T3 cells (Cheng etal., 1997), and introduction of Akt antisense RNA inhibits the tumorigenic phenotype of cancer cells expressing high levels of Akt (Cheng etal., 1996). The IGF/Akt pathway is probably also important in regulating cell size, as suggested by the phenotypes of PTEN (a phosphatase that inhibits the Akt pathway) (reviewed in Backman etal., 2002), and thus plays a critical role in regulating long-term proliferation, and its expression is correlated with enhanced lifespan. IGF and insulin are ubiquitously expressed and appear to be important in early development of many tissues. Cardiac development and growth depend upon activation of Akt (Klinz etal., 1999). Hepatic and pancreatic development requires IGF signaling, and aberrant signaling such as in PTEN mutants leads to early embryonic abnormalities. Thus, Akt may play an important role in regulating cellular senescence and cell death in ES cells. Recent studies have also shown that IGF-1 plays an important role in preventing apoptosis in the early development of the embryo, as well as in the progressive regulation of organ development (Lin etal., 2003). IGF-1 signaling is known to be mediated via IGF-1 receptor (O’Connor etal., 1997; Prisco etal., 1999; Allan etal., 2000), which is expressed during early blastocyst development. The PI3 kinase/Akt signaling pathway has been implicated in ES cell self-renewal through studies of ES cells lacking PTEN (Sun etal., 1999; Crackower etal., 2002). These PTEN knockout ES cells have both increased viability and an increased rate of cell proliferation. Importantly, Pten–/– mouse embryo fibroblasts (MEF) also up-regulated and activated the Akt-controlled anti-cell death pathway (Sun etal., 1999). However, the increase in the rate of cell division is more marked in Pten–/– ES cells than in Pten–/– MEF cells, highlighting the importance of this pathway in ES cell self-renewal. These findings suggest that the PI3K/Akt pathway might influence ES cell growth through a pathway that is dependent on IGF-1. However, it still remains unclear whether the IGF/PI3K/Akt pathway plays an important role in ES cell self-renewal. Examining expression of this pathway in human ES cells and comparing levels with expression in EB (Table3) suggest that this pathway is active in both ES cells and EB, and, somewhat surprisingly, that the gene expressions of the IGF/PI3K/Akt pathway were relatively high in EB compared with ES cells [for example, IGF1R (ES:EB=14:32), IRS1 (1:52), PDK2 (0:15), ILK (12:139) and Akt (75:157)]. Consistent with this result, PTEN is expressed at higher levels in ES cells than in EB [PTEN (25:6)]. Indeed, it has also been reported that FGF signaling through PI3K/Akt is required for mouse embryoid body differentiation (Chen etal., 2000). Thus, these MPSS data suggest that IGF/PI3K/Akt pathway genes may be differently regulated in mouse and human cells, and that these differences may be important in understanding the differences in ES cell cycle and self-renewal. The abundance for each listed gene was converted to transcripts per million (Tpm) for the purpose of comparison between human ES cells and EB. This review discusses mechanisms of immortalization and/or self-renewal in ES cells and suggests that these are tightly shared with cellular senescence pathways (Fig.4 and Table4). We suggest that although speculative, this review provides a framework to test specific hypotheses and select appropriate readouts. ES cell lines offer a useful model to study such processes in a non-transformed spontaneously immortal cell line. Importantly, because ES cells subsequently differentiate to generate cells that will senesce, ES cell lines offer a unique model system to examine the transition from a non-senescent to senescent state. As over 100 ES cell lines are available, allelic variability in the aging process can begin to be studied in an in vitro model and perturbation techniques can be readily adapted to examine senescence. Furthermore, we believe that antagonizing the senescence pathway or growth arrest pathway in ES cells may be one key factor required to achieve constitutive proliferation. We suggest that studies of senescence will help ES biologists as well by identifying key regulators for karyotypic stability and prolonged self-renewal. Overview of selected elements that influence self-renewal and differentiation in ES cells. Asterisks indicate those pathways that are predicted to regulate the self-renewal and differentiation in ES cells based on current views (see also Table4). This research was supported by the National Institutes on Health and the CNS Foundation to M.S.R. T.M. was supported by the NIA. We gratefully acknowledge the input of all members of our laboratory provided through discussions and constructive criticisms. M.S.R. acknowledges the contributions of Dr S. Rao that made undertaking this project possible.Jaenisch R, Wahl GM (1998) ES cells do not activate p53-dependent stress responses and undergo p53-independent apoptosis in response to DNA damage. Curr. Biol. 8, 145– 155.Geh J, Patel K (2000) Altered expression of insulin-like growth factor-1 and insulin like growth factor binding proteins-2 and 5 in the mouse mutant Hypodactyly (Hd) correlates with sites of apoptotic activity. Anat. Embryol. (Berl.) 202, 1– 11.Cairns PM, Hole N (2000) mTert expression correlates with telomerase activity during the differentiation of murine embryonic stem cells. Mech. Dev. 97, 109– 116. Artandi SE, DePinho RA (2000) Mice without telomerase: what can they teach us about human cancer? Nat. Med. 6, 852– 855. Baumann P, Cech TR (2001) Pot1, the putative telomere end-binding protein in fission yeast and humans. Science 292, 1171– 1175.Podell E, Cech TR (2002) Human Pot1 (protection of telomeres) protein: cytolocalization, gene structure, and alternative splicing. Mol. Cell Biol. 22, 8079– 8087.Smith JR, Pereira-Smith OM (1999) Identification of a gene that reverses the immortal phenotype of a subset of cells and is a member of a novel family of transcription factor-like genes. Mol. Cell Biol. 19, 1479– 1485.Rao MS, Puri RK (2004) Gene expression in human embryonic stem cell lines: unique molecular signature. Blood 103, 2956– 2964.DePinho RA, Greider CW (1997) Telomere shortening and tumor formation by mouse cells lacking telomerase RNA. Cell 91, 25– 34.Lebkowski J, Stanton LW (2004b) Transcriptome characterization elucidates signaling networks that control human ES cell growth and differentiation. Nat. Biotechnol. 22, 707– 716.Chong L, De Lange T (1997) Human telomeres contain two distinct Myb-related proteins, TRF1 and TRF2. Nat. Genet. 17, 231– 235.Smith A, Savatier P (2002) signaling, cell cycle and pluripotency in embryonic stem cells. Trends Cell Biol. 12, 432– 438. Campisi J (2001) Cellular senescence as a tumor-suppressor mechanism. Trends Cell Biol. 11, S27– S31.Chen CY, Cheng AJ (2002) Differential regulation of telomerase activity by six telomerase subunits. Eur. J. Biochem. 269, 3442– 3450.Seger R, Lonai P (2000) Fibroblast growth factor (FGF) signaling through PI 3-kinase and Akt/PKB is required for embryoid body differentiation. Oncogene 19, 3750– 3756.Lissy NA, Testa JR (1997) Transforming activity and mitosis-related expression of the AKT2 oncogene: evidence suggesting a link between cell cycle regulation and oncogenesis. Oncogene 14, 2793– 2801.Watson DK, Testa JR (1996) Amplification of AKT2 in human pancreatic cells and inhibition of AKT2 expression and tumorigenicity by antisense RNA. Proc. Natl Acad. Sci. USA 93, 3636– 3641. Cole MD, McMahon SB (1999) The Myc oncoprotein: a critical evaluation of transactivation and target gene regulation. Oncogene 18, 2916– 2924.Reddel RR (2003) Human POT1 facilitates telomere elongation by telomerase. Curr. Biol. 13, 942– 946.Wen J, Bacchetti S (1999) The human telomerase catalytic subunit hTERT: organization of the gene and characterization of the promoter. Hum. Mol. Genet. 8, 137– 142.Backx PH, Penninger JM (2002) Regulation of myocardial contractility and cell size by distinct PI3K-PTEN signaling pathways. Cell 110, 737– 749. Davis A, Bradley A (1993) Mutation of N-myc in mice: what does the phenotype tell us? Bioessays 15, 273– 275.Hann SR, Bradley A (1993) A null c-myc mutation causes lethality before 10.5 days of gestation in homozygotes and reduced fertility in heterozygous female mice. Genes Dev. 7, 671– 682.Clarkin K, Wahl GM (1994) DNA damage triggers a prolonged p53-dependent G1 arrest and long-term induction of Cip1 in normal human fibroblasts. Genes Dev. 8, 2540– 2551.Acosta M, Campisi J (2000) Regulation of a senescence checkpoint response by the E2F1 transcription factor and p14 (ARF) tumor suppressor. Mol. Cell Biol. 20, 273– 285. Butel JS, Bradley A (1992) Mice deficient for p53 are developmentally normal but susceptible to spontaneous tumours. Nature 356, 215– 221.Hannon GJ, Lowe SW (2002) Oncogenic ras and p53 cooperate to induce cellular senescence. Mol. Cell Biol. 22, 3497– 3508.Wright WE, Shay JW (2002) Telomerase and differentiation in multicellular organisms: turn it off, turn it on, and turn it off again. Differentiation 69, 188– 197.Itskovitz-Eldor J, Rao MS (2004) Differences between human and mouse embryonic stem cells. Dev. Biol. 269, 360– 380.Haulon S, Nolte L (2004) Analysis of renal function after aneurysm repair with a device using suprarenal fixation (Zenith AAA Endovascular Graft) in contrast to open surgical repair. J. Vasc. Surg. 39, 1219– 1228.Morin GB, DePinho RA (1999) Telomerase reverse transcriptase gene is a direct target of c-Myc but is not functionally equivalent in cellular transformation. Oncogene 18, 1219– 1226. Greider CW, Blackburn EH (1987) The telomere terminal transferase of Tetrahymena is a ribonucleoprotein enzyme with two kinds of primer specificity. Cell 51, 887– 898. Halaban R (1999) Melanoma cell autonomous growth: the Rb/E2F pathway. Cancer Metastasis Rev. 18, 333– 343.Moorhead PS (1961) The serial cultivation of human diploid cell strains. Exp. Cell Res. 25, 585– 621.Tanaka H, Yasuda H (1997) Oncoprotein MDM2 is a ubiquitin ligase E3 for tumor suppressor p53. FEBS Lett. 420, 25– 27.Afshari C, Barrett JC (1999) Cloning and characterization of the promoter region of human telomerase reverse transcriptase gene. Cancer Res. 59, 826– 830.Lanzendorf SE, Picton HM (2004) Expression of mRNAs for DNA methyltransferases and methyl-CpG-binding proteins in the human female germ line, preimplantation embryos, and embryonic stem cells. Mol. Reprod. Dev. 67, 323– 336.Markossian S, Savatier P (2002) Differential contributions of ERK and PI3-kinase to the regulation of cyclin D1 expression and to the control of the G1/S transition in mouse embryonic stem cells. Oncogene 21, 5515– 5528.Donehower LA, Bradley A (1995) Rescue of embryonic lethality in Mdm2-deficient mice by absence of p53. Nature 378, 206– 208.Ravindranath Y, Craig RW (1991) Levels of p53 protein increase with maturation in human hematopoietic cells. Cancer Res. 51, 4279– 4286.Miura T, Shirahata S (1999) Transforming growth factor beta triggers two independent-senescence programs in cancer cells. Biochem. Biophys. Res. Commun. 255, 110– 115.Tsuchiya T, Shirahata S (2003) Decreased tumorigenicity in vivo when transforming growth factor beta treatment causes cancer cell senescence. Biosci. Biotechnol. Biochem. 67, 815– 821.Weinrich SL, Shay JW (1994) Specific association of human telomerase activity with immortal cells and cancer. Science 266, 2011– 2015.Galloway DA, Klingelhutz AJ (1998) Both Rb/p16INK4a inactivation and telomerase activity are required to immortalize human epithelial cells. Nature 396, 84– 88.Addicks K, Hescheler J (1999) Inhibition of phosphatidylinositol-3-kinase blocks development of functional embryonic cardiomyocytes. Exp. Cell Res. 247, 79– 83.Rao KS, Bohr VA (1996) Telomere reduction and telomerase inactivation during neuronal cell differentiation. Biochem. Biophys. Res. Commun. 224, 487– 492.Luscher TF, Erusalimsky JD (2003) Fibroblast growth factor-2, but not vascular endothelial growth factor, upregulates telomerase activity in human endothelial cells. Arterioscler. Thromb. Vasc. Biol. 23, 748– 754.Ariga H, Inoue M (2000) Sp1 cooperates with c-Myc to activate transcription of the human telomerase reverse transcriptase gene (hTERT). Nucleic Acids Res. 28, 669– 677.Pelicci PG, Kouzarides T (2002) Human SIR2 deacetylates p53 and antagonizes PML/p53-induced cellular senescence. EMBO J. 21, 2383– 2396.Lu KP, Aaronson SA (2000) Sustained activation of Ras/Raf/mitogen-activated protein kinase cascade by the tumor suppressor p53. Proc. Natl Acad. Sci. USA 97, 8302– 8305.Hart SR, Skalnik DG (2004) Histone deacetylase activity is required for embryonic stem cell differentiation. Genesis 38, 32– 38.Serrano M, Lowe SW (1998) Premature senescence involving p53 and p16 is activated in response to constitutive MEK/MAPK mitogenic signaling. Genes Dev. 12, 3008– 3019. Lin SY, Elledge SJ (2003) Multiple tumor suppressor pathways negatively regulate telomerase. Cell 113, 881– 889.Libina N, Kenyon C (2001) Regulation of the Caenorhabditis elegans longevity protein DAF-16 by insulin/IGF-1 and germline signaling. Nat. Genet. 28, 139– 145.Hsu TT, Chen LR (2003) IGF-1/IGFBP-1 increases blastocyst formation and total blastocyst cell number in mouse embryo culture and facilitates the establishment of a stem-cell line. BMC Cell Biol. 4, 14. Loayza D, De Lange T (2003) POT1 as a terminal transducer of TRF1 telomere length control. Nature 423, 1013– 1018. Longo VD, Finch CE (2003) Evolutionary medicine: from dwarf model systems to healthy centenarians? Science 299, 1342– 1346.Andrews LG, Tollefsbol TO (2003) Control mechanisms in the regulation of telomerase reverse transcriptase expression in differentiating human teratocarcinoma cells. Biochem. Biophys. Res. Commun. 306, 650– 659.May P, Mora PT (1988) The phosphoprotein p53 is down-regulated post-transcriptionally during embryogenesis in vertebrates. Biochim. Biophys. Acta 950, 395– 402.Lane DP, Hall PA (1996) The p53 response to ionising radiation in adult and developing murine tissues. Oncogene 13, 2575– 2587.Carpenter MK, Rao MS (2004) Monitoring early differentiation events in human embryonic stem cells by MPSS and EST scan. Stem Cells Dev. in press.Toko H, Komuro I (2004) Akt negatively regulates the in vitro lifespan of human endothelial cells via a p53/p21-dependent pathway. EMBO J. 23, 212– 220.Wagner DS, Lozano G (1995) Rescue of early embryonic lethality in mdm2-deficient mice by deletion of p53. Nature 378, 203– 206.Furuichi Y, Shinkai Y (1998) Severe growth defect in mouse cells lacking the telomerase RNA component. Nat. Genet. 19, 203– 206.Baserga R, Blattler WA (1997) Identification of domains of the insulin-like growth factor I receptor that are required for protection from apoptosis. Mol. Cell Biol. 17, 427– 435.Reich NC, Levine AJ (1982) Regulation of the cellular p53 tumor antigen in teratocarcinoma cells and their differentiated progeny. Mol. Cell Biol. 2, 443– 449.Malumbres M, Barbacid M (2002) Cyclin d-dependent kinases, INK4 inhibitors and cancer. Biochim. Biophys. Acta 1602, 73– 87.Pandolfi PP, Pelicci PG (2000) PML regulates p53 acetylation and premature senescence induced by oncogenic Ras. Nature 406, 207– 210.Valentinis B, Baserga R (1999) Insulin and IGF-I receptors signaling in protection from apoptosis. Horm. Metab. Res. 31, 80– 89.Wyllie AH, Harrison DJ (1998) p53-independent DNA repair and cell cycle arrest in embryonic stem cells. FEBS Lett. 425, 499– 504.Rao MS, Carpenter MK (2004) Long-term culture of human embryonic stem cells in feeder-free conditions. Dev. Dyn. 229, 259– 274.Naef F, Brivanlou AH (2003) Molecular signature of human embryonic stem cells and its comparison with the mouse. Dev. Biol. 260, 404– 413.Wiman KG, Samarut J (1994) Contrasting patterns of retinoblastoma protein expression in mouse embryonic stem cells and embryonic fibroblasts. Oncogene 9, 809– 818.Rudkin BB, Samarut J (1996) Withdrawal of differentiation inhibitory activity/leukemia inhibitory factor up-regulates d-type cyclins and cyclin-dependent kinase inhibitors in mouse embryonic stem cells. Oncogene 12, 309– 322.Beach D, Lowe SW (1997) Oncogenic ras provokes premature cell senescence associated with accumulation of p53 and p16INK4a. Cell 88, 593– 602. Smith JR, Pereira-Smith OM (1996) Replicative senescence: implications for in vivo aging and tumor suppression. Science 273, 63– 67.Crescenzi M, Sacchi A (1996) Interference with p53 protein inhibits hematopoietic and muscle differentiation. J. Cell Biol. 134, 193– 204.Brown PO, Thomson JA (2003) Gene expression patterns in human embryonic stem cells and human pluripotent germ cell tumors. Proc. Natl Acad. Sci. USA 100, 13350– 13355.De Lange T, Griffith JD (2001) T-loop assembly in vitro involves binding of TRF2 near the 3′ telomeric overhang. EMBO J. 20, 5532– 5540.Woodgett JR, Manoukian AS (1998) Genetic analysis of protein kinase B (AKT) in Drosophila. Curr. Biol. 8, 599– 602.Walker D, Dalton S (2002) Pluripotent cell division cycles are driven by ectopic Cdk2, cyclin A/E and E2F activities. Oncogene 21, 8320– 8333. Van Steensel B, De Lange T (1997) Control of telomere length by the human telomeric protein TRF1. Nature 385, 740– 743.Smogorzewska A, De Lange T (1998) TRF2 protects human telomeres from end-to-end fusions. Cell 92, 401– 413.Liu X, Wu H (1999) PTEN modulates cell cycle progression and cell survival by regulating phosphatidylinositol 3,4,5,-trisphosphate and Akt/protein kinase B signaling pathway. Proc. Natl Acad. Sci. USA 96, 6199– 6204.Yutsudo M, Inoue M (1999) Cloning of human telomerase catalytic subunit (hTERT) gene promoter and identification of proximal core promoter sequences essential for transcriptional activation in immortalized and cancer cells. Cancer Res. 59, 551– 557.Lloyd AC, Raff MC (2001) Lack of replicative senescence in cultured rat oligodendrocyte precursor cells. Science 291, 868– 871.Bellacosa A (2001) AKT plays a central role in tumorigenesis. Proc. Natl Acad. Sci. USA 98, 10983– 10985.Marshall VS, Jones JM (1998) Embryonic stem cell lines derived from human blastocysts. Science 282, 1145– 1147.Bradley A, Donehower LA (2002) p53 mutant mice that display early ageing-associated phenotypes. Nature 415, 45– 53. Van Zant G (2003) Genetic control of stem cells: implications for aging. Int. J. Hematol. 77, 29– 36. Vanfleteren JR, Braeckman BP (1999) Mechanisms of life span determination in Caenorhabditis elegans. Neurobiol. Aging 20, 487– 502.Rotter V (1997) The DNA binding regulatory domain of p53: see the C. Pathol Biol. (Paris) 45, 785– 796. Wright WE, Shay JW (2000) Telomere dynamics in cancer progression and prevention: fundamental differences in human and mouse telomere biology. Nat. Med. 6, 849– 851.Zwaka TP, Thomson JA (2002) BMP4 initiates human embryonic stem cell differentiation to trophoblast. Nat. Biotechnol. 20, 1261– 1264.Gold JD, Carpenter MK (2001) Feeder-free growth of undifferentiated human embryonic stem cells. Nat. Biotechnol. 19, 971– 974.Hsu HC, Mountz JD (2004) Regulation of apoptosis proteins in cancer cells by ubiquitin. Oncogene 23, 2009– 2015. The full text of this article hosted at iucr.org is unavailable due to technical difficulties. Please check your email for instructions on resetting your password. If you do not receive an email within 10 minutes, your email address may not be registered, and you may need to create a new Wiley Online Library account. Can\'t sign in? Forgot your username? Enter your email address below and we will send you your username If the address matches an existing account you will receive an email with instructions to retrieve your username
本文链接: https://www.ebiomall.cn/b215-origene/info-1078564387.html
免责声明 本文仅代表作者个人观点,与本网无关。其创作性以及文中陈述文字和内容未经本站证实,对本文以及其中全部或者部分内容、文字的真实性、完整性、及时性本站不做任何保证或承诺,请读者仅作参考,并请自行核实相关内容。
版权声明 未经蚂蚁淘授权不得转载、摘编或利用其他方式使用上述作品。已经经本网授权使用作品的,应该授权范围内使用,并注明“来源:蚂蚁淘”。违反上述声明者,本网将追究其相关法律责任。
相关文章
1970-01-01
1970-01-01
2011-07-01
2012-08-31
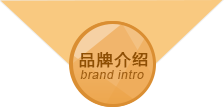
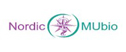
▍
品牌分类
▍
官网分类