新闻动态
Particle size distribution of exosomes and microvesicles...
2025-07-16
Particle size distribution of exosomes and microvesicles determined by transmission electron microscopy, flow cytometry, nanoparticle tracking analysis, and resistive pulse sensing - Pol - 2014 - Journal of Thrombosis and Haemostasis - Wiley Online LibraryJournal of Thrombosis and HaemostasisVolume 12, Issue 7 p. 1182-1192 Original Article Free Access Particle size distribution of exosomes and microvesicles determined by transmission electron microscopy, flow cytometry, nanoparticle tracking analysis, and resistive pulse sensing E. van der Pol, Corresponding Author Laboratory of Experimental Clinical Chemistry, Academic Medical Center, University of Amsterdam, Amsterdam, The Netherlands Biomedical Engineering and Physics, Academic Medical Center, University of Amsterdam, Amsterdam, the Netherlands Correspondence: Edwin van der Pol, Biomedical Engineering and Physics, Academic Medical Center, University of Amsterdam, Meibergdreef 9, PO Box 22660, 1100 DD, Amsterdam, the Netherlands. Tel.: +31 20 5664386; fax: +31 20 5669569. E-mail: e.vanderpol@amc.uva.nlSearch for more papers by this authorF. A. W. Coumans, Laboratory of Experimental Clinical Chemistry, Academic Medical Center, University of Amsterdam, Amsterdam, The Netherlands Biomedical Engineering and Physics, Academic Medical Center, University of Amsterdam, Amsterdam, the NetherlandsSearch for more papers by this authorA. E. Grootemaat, Laboratory of Experimental Clinical Chemistry, Academic Medical Center, University of Amsterdam, Amsterdam, The NetherlandsSearch for more papers by this authorC. Gardiner, Nuffield Department of Obstetrics and Gynaecology, John Radcliffe Hospital, Oxford, UKSearch for more papers by this authorI. L. Sargent, Nuffield Department of Obstetrics and Gynaecology, John Radcliffe Hospital, Oxford, UKSearch for more papers by this authorP. Harrison, School of Immunity and Infection, University of Birmingham Medical School, Birmingham, UKSearch for more papers by this authorA. Sturk, Laboratory of Experimental Clinical Chemistry, Academic Medical Center, University of Amsterdam, Amsterdam, The NetherlandsSearch for more papers by this authorT. G. van Leeuwen, Biomedical Engineering and Physics, Academic Medical Center, University of Amsterdam, Amsterdam, the NetherlandsSearch for more papers by this authorR. Nieuwland, Laboratory of Experimental Clinical Chemistry, Academic Medical Center, University of Amsterdam, Amsterdam, The NetherlandsSearch for more papers by this author E. van der Pol, Corresponding Author Laboratory of Experimental Clinical Chemistry, Academic Medical Center, University of Amsterdam, Amsterdam, The Netherlands Biomedical Engineering and Physics, Academic Medical Center, University of Amsterdam, Amsterdam, the Netherlands Correspondence: Edwin van der Pol, Biomedical Engineering and Physics, Academic Medical Center, University of Amsterdam, Meibergdreef 9, PO Box 22660, 1100 DD, Amsterdam, the Netherlands. Tel.: +31 20 5664386; fax: +31 20 5669569. E-mail: e.vanderpol@amc.uva.nlSearch for more papers by this authorF. A. W. Coumans, Laboratory of Experimental Clinical Chemistry, Academic Medical Center, University of Amsterdam, Amsterdam, The Netherlands Biomedical Engineering and Physics, Academic Medical Center, University of Amsterdam, Amsterdam, the NetherlandsSearch for more papers by this authorA. E. Grootemaat, Laboratory of Experimental Clinical Chemistry, Academic Medical Center, University of Amsterdam, Amsterdam, The NetherlandsSearch for more papers by this authorC. Gardiner, Nuffield Department of Obstetrics and Gynaecology, John Radcliffe Hospital, Oxford, UKSearch for more papers by this authorI. L. Sargent, Nuffield Department of Obstetrics and Gynaecology, John Radcliffe Hospital, Oxford, UKSearch for more papers by this authorP. Harrison, School of Immunity and Infection, University of Birmingham Medical School, Birmingham, UKSearch for more papers by this authorA. Sturk, Laboratory of Experimental Clinical Chemistry, Academic Medical Center, University of Amsterdam, Amsterdam, The NetherlandsSearch for more papers by this authorT. G. van Leeuwen, Biomedical Engineering and Physics, Academic Medical Center, University of Amsterdam, Amsterdam, the NetherlandsSearch for more papers by this authorR. Nieuwland, Laboratory of Experimental Clinical Chemistry, Academic Medical Center, University of Amsterdam, Amsterdam, The NetherlandsSearch for more papers by this author Give accessShare full text accessShare full-text accessPlease review our Terms and Conditions of Use and check box below to share full-text version of article.I have read and accept the Wiley Online Library Terms and Conditions of UseShareable LinkUse the link below to share a full-text version of this article with your friends and colleagues. Learn more.Copy URLShare a linkShare onEmailFacebookTwitterLinked InRedditWechat Summary Background Enumeration of extracellular vesicles has clinical potential as a biomarker for disease. In biological samples, the smallest and largest vesicles typically differ 25-fold in size, 300000-fold in concentration, 20000-fold in volume, and 10000000-fold in scattered light. Because of this heterogeneity, the currently employed techniques detect concentrations ranging from 104 to 1012vesiclesmL–1.Objectives To investigate whether the large variation in the detected concentration of vesicles is caused by the minimum detectable vesicle size of five widely used techniques. Methods The size and concentration of vesicles and reference beads were measured with transmission electron microscopy (TEM), a conventional flow cytometer, a flow cytometer dedicated to detecting submicrometer particles, nanoparticle tracking analysis (NTA), and resistive pulse sensing (RPS). Results Each technique gave a different size distribution and a different concentration for the same vesicle sample. Conclusion Differences between the detected vesicle concentrations are primarily caused by differences between the minimum detectable vesicle sizes. The minimum detectable vesicle sizes were 70–90nm for NTA, 70–100nm for RPS, 150–190nm for dedicated flow cytometry, and 270–600nm for conventional flow cytometry. TEM could detect the smallest vesicles present, albeit after adhesion on a surface. Dedicated flow cytometry was most accurate in determining the size of reference beads, but is expected to be less accurate on vesicles, owing to heterogeneity of the refractive index of vesicles. Nevertheless, dedicated flow cytometry is relatively fast and allows multiplex fluorescence detection, making it most applicable to clinical research. Introduction Extracellular vesicles, such as exosomes and microvesicles, are released by cells into their environment as submicrometer particles enclosed by a phospholipid bilayer 1. These vesicles contribute to many homeostatic processes, e.g. coagulation and inflammation 2-4, and therefore have potential clinical applications 5-8. Unfortunately, most single vesicles are below the detection range of many techniques, owing to their small size and low refractive index 9, 10, leading to misinterpretation of data and reported concentrations ranging from 104 to 1012vesiclesmL–1 in plasma 9-15. In 2010, we reviewed the theoretical performance of 13 methods to determine the particle size distribution (PSD) of vesicles 9. The PSD describes the concentration as a function of size, and defines which vesicle types are measured 4. Unexpectedly, our simulations predicted that each method would obtain a different PSD, thereby hampering data interpretation, data comparison, and standardization. In this study, we performed an experimental evaluation of five of the 13 methods. We selected the most widely used methods capable of detecting single vesicles: transmission electron microscopy (TEM), a conventional flow cytometer, a flow cytometer dedicated to detecting submicrometer particles, nanoparticle tracking analysis (NTA), and resistive pulse sensing (RPS). The PSDs of a standard population of reference beads and a standard population of vesicles were measured with all methods. Throughout this article, we define ‘size’ as the diameter of a particle, and the PSD as the histogram of particle sizes, providing the mean number of particles per milliliter per 10-nm bin 16. Data processing and representation were performed with originpro (v8.0724; OriginLab Corporation, Northampton, MA, USA). To create a reference sample with a known PSD, a mixture of traceable polystyrene beads (Nanosphere; Thermo Fisher, Waltham, MA, USA) was prepared in de-ionized water. RPS measurements require a conductive medium; therefore, the beads were suspended in electrolyte buffer (Izon, Christchurch, New Zealand). The size and concentration of the reference beads were selected to resemble those of previously reported vesicle PSDs 9, 11-13, 15, 17. Table1 shows the size of the reference beads according to TEM data of the manufacturer. The concentration (beadsmL−1) was derived from the specifications. Figure1A shows the PSD of the reference beads under the assumption that each subpopulation has a Gaussian distribution. The reference sample contained five subpopulations, among which larger beads have lower concentrations. The total concentration was 3.1×109 beads mL−1. Prior to analysis, the reference sample was sonicated for 10s and vortexed for 10s. Table2 lists the diameters of silica beads (Silica Oxide Size Standards [Corpuscular, Cold Spring, NY, USA]; Plain Silica [Kisker, Steinfurt, Germany]) used to calibrate the flow cytometers and NTA instrument. Table 1. Catalog numbers, diameters and concentrations of the subpopulations of polystyrene reference beads as determined by five methods NTA, nanoparticle tracking analysis; RPS, resistive pulse sensing; TEM, transmission electron microscopy. Diameter is expressed as mean±standard deviation. Table 2. Manufacturers, catalog numbers and diameters of silica beads obtained by imaging at least 500 beads with transmission electron microscopy Figure 1Open in figure viewerPowerPoint Particle size distribution (PSD). Concentrations (on a logarithmic scale) of the reference beads (left) and the vesicle standard (right) detected by (A, B) transmission electron microscopy (TEM), (C, D) conventional flow cytometry, (E, F) dedicated flow cytometry, (G, H) nanoparticle tracking analysis (NTA) and (I, J) resistive pulse sensing (RPS) are shown. The bin width is 10nm. PSDs of the reference beads (black line) were fitted by a sum of Gaussian functions (dotted red line). PSDs of the vesicle standard (black line) were fitted by a power-law function (dashed green line). The PSD of the reference beads determined by TEM is based on data from the manufacturer. PSDs given by NTA and RPS originate from two measurements with relatively high-sensitivity (black) and low-sensitivity (blue) settings. As isolation of vesicles from blood is challenging 18, we selected urinary vesicles for our biological standard sample. Urine contains a relatively high concentration of vesicles with low contamination 9. Urine from five healthy male individuals was collected, pooled, and centrifuged twice (8×50mL, 10min, 180×g, 4°C; and 20min, 1550×g) to remove cells. Cell-free urine aliquots (12mL) were frozen in liquid nitrogen and stored at –80°C. Prior to analysis, samples were thawed on melting ice for 1h, centrifuged (10min, 1550×g, 4°C) to remove precipitated salts, and diluted in 0.2-μm-filtered (MilliPore, Billerica, MA, USA) phosphate-buffered saline. Data from the manufacturer were used to create the PSD of reference beads by TEM. For analysis of the vesicle standard by TEM (CM-10; Philips, Eindhoven, The Netherlands), vesicles were prepared and analyzed as described in DataS1. To obtain the vesicle concentration, we multiplied the mean number of vesicles per surface area by the grid area, divided by the sample volume. Here, we assumed that all vesicles adhered to the grid and were distributed uniformly. A flow cytometer (FACSCalibur; BD, Franklin Lakes, NJ, USA) with a 15-mW 488-nm laser was used to detect side-scattered light (SSC) for 10min at a flow rate of ~60μLmin−1. The detector settings are described in DataS1. To calculate the particle concentration, the flow rate was determined by weighting the sample volume aspirated during 10min. To prevent swarm detection 15, the reference beads and vesicle standard were diluted 1000-fold (1.7×105counts vs. 1.0×105background counts) and 100-fold (2.6×105counts vs. 1.5×105 background counts), respectively. The absence of swarm detection was confirmed by serial dilutions. To relate SSC to a particle size, we calibrated the flow cytometer with beads of known size and refractive index. Figure2A shows the SSC histogram of polystyrene beads. Figure2B shows the SSC of polystyrene and silica beads vs. their size. The data were fitted by Mie theory, incorporating the size and refractive index of the beads and the optical configuration of the instrument 19. Mie calculations were performed with the scripts of Mätzler 20 in matlab (v7.9.0.529). The solid curve in Fig.2B was used to relate SSC to the size of the polystyrene reference beads with a refractive index of 1.61 15. The dashed curve in Fig.2B was used to relate SSC to vesicle size, with the assumption that vesicles are spheres with a refractive index of 1.40, which was previously estimated 14 and corresponds to the refractive index of cells 21, 22. Figure 2Open in figure viewerPowerPoint Relationship between scattering and the diameter of vesicles. (A) Side-scattered light (SSC; logarithmic scale) vs. concentration for polystyrene beads measured by conventional flow cytometry. (B) Measured (symbols) and calculated (lines) SSC (logarithmic scale) vs. diameter for polystyrene beads (black), silica beads (red), and vesicles (green). The SSC increases with increasing particle diameter, and is lower for vesicles than for beads. (C) Forward-scattered light (FSC; logarithmic scale) vs. concentration for polystyrene beads measured by dedicated flow cytometry. The concentration of 102-nm beads was 100-fold higher than that of the other sizes to discriminate between signal and background counts. (D) Measured (symbols) and calculated (lines) FSC (logarithmic scale) vs. diameter for polystyrene beads (black), silica beads (red), and vesicles (green). Mie calculations are in excellent agreement with the data, except for the 799-nm and 994-nm polystyrene beads, owing to detector saturation. a.u., arbitrary units. Throughout this article, we use ‘dedicated flow cytometry’ as a generic term for flow cytometers dedicated to detecting submicrometer particles. A flow cytometer (A50-Micro; Apogee, Hemel Hempstead, UK) with a 20-mW 488-nm laser was used to detect forward-scattered light (FSC) and SSC. The detector settings are described in DataS1. The sample volume injected by the internal microsyringe was used to calculate the concentration of particles. In total, 1.4×105 reference beads and 0.8×105 vesicles were analyzed. Analogously to our approach for conventional flow cytometry, we related FSC to the vesicle size by using beads and Mie theory, as illustrated for dedicated flow cytometry in Fig.2C,D. A dark-field microscope (NS500; Nanosight, Amesbury, UK) with a 45-mW 405-nm laser and an electron multiplying charge-coupled device (EMCCD) was used to determine the PSD by tracking the Brownian motion of single particles 12, 23. Measurements were performed with two dilutions and two detection settings to increase the effective size range, which is needed because light scattered from the smallest and the largest beads differs by five orders of magnitude, whereas the dynamic range of the EMCCD is only approximately three orders of magnitude. Consequently, settings suitable for detecting 46-nm beads would result in extreme saturation for 596-nm beads. Two dilutions are needed, because a 50-fold dilution is required to detect the smallest beads 23, but at this dilution the probability of detecting a 596-nm bead is 0.5%. Reference beads were analyzed with high-sensitivity settings (diluted 1:50; shutter, 26.67ms; gain, 650; threshold, 22; 1.8×103 beads tracked) and low-sensitivity settings (undiluted; shutter, 1.67ms; gain, 100; threshold, 10; 1.1×104 beads tracked). We multiplied the concentration as provided by the NTA software by the ratio between the expected and measured concentrations of calibration beads 23. This concentration calibration was performed with 102-nm and 203-nm polystyrene beads with concentrations of 2×107 and 1×108beadsmL−1 for the high-sensitivity and low-sensitivity settings, respectively. The vesicle standard was analyzed with high-sensitivity settings (diluted 1:500; shutter, 26.67ms; gain, 650; threshold, 19; 1.0×103 vesicles tracked) and low-sensitivity settings (diluted 1:100; shutter, 26.67ms; gain, 400; threshold, 10; 1.1×103 vesicles tracked). Concentration calibration was performed with 105-nm and 206-nm silica beads with a concentration of 1×108 beadsmL−1 for both the high-sensitivity and low-sensitivity settings 23, as the refractive indices of silica and vesicles are close. Per sample, 20 videos of 30s were captured at 22.0°C and analyzed by NTA v2.3.0.17 (Nanosight), assuming a medium viscosity of 0.95cP. To obtain the overall PSD O(d), the PSDs obtained with high-sensitivity settings, H(d), and low-sensitivity settings, L(d), were combined at the size d0, where the concentrations were similar [H(d0)≈L(d0), O(d)=H(d) for all d≤d0; O(d)=L(d) for all d d0]. RPS (qNano; Izon) determines the PSD from resistance pulses caused by particles moving through a pore. Measurements were performed with two pore sizes, for two reasons. First, for a single pore, the detectable size range is at best five-fold, whereas our smallest and largest reference beads differ 12-fold in size. Second, the analyzed sample volume depends on the pore size. With the NP100 pore, only 0.9nL of sample was analyzed, containing 1 bead of 596nm on average. With the NP400 pore, 80nL was analyzed, containing 77 beads of 596nm. The reference beads were analyzed by RPS with high-sensitivity settings (NP100; voltage, 0.70V; stretch, 47.0mm) and low-sensitivity settings (NP400; voltage, 0.26V; stretch, 46.5mm). The vesicle standard was diluted 1:1, and analyzed with high-sensitivity settings (NP100; voltage, 0.60V; stretch, 46.0mm) and low-sensitivity settings (NP400; voltage, 0.40V; stretch, 43.5mm). The pressure was set at 7.0mbar. Calibration was performed with beads supplied by the manufacturer. At least 1000 particles per sample were analyzed. A custom-made Microsoft Visual Basic 2008 application interrupted the measurement when the root-mean-square noise exceeded 10pA. We required the R2-correlation of cumulative counts with time to exceed 0.999, and the baseline current drift not to exceed 5%. The two PSDs are combined in a similar way as for NTA. Figure1 shows all PSDs of the reference beads and vesicle standard. Figure1A shows the reference bead PSD based on the prepared concentrations and manufacturer-supplied data. Figure1B shows the vesicle standard PSD measured by TEM. The combined PSD has a peak at 45nm, and for larger vesicles the concentration decreases with increasing size. The spikes on the right-hand side correspond to single vesicles. TEM can detect the smallest vesicles present, owing to an imaging resolution of ~1nm. However, sample preparation may cause a reduction in vesicle size 18, 24, 25. In addition, limited and non-uniform adhesion of vesicles on the surface may affect the PSD. Figure1C shows that the smallest polystyrene bead detected by conventional flow cytometry was 203nm, and that the peaks were broadened as compared with the reference bead PSD. Figure1D shows that the first bin of the vesicle standard PSD corresponds to 340nm, which is 140nm larger than the smallest detected polystyrene bead, owing to refractive index differences. The detected concentration was 1.8×107vesiclesmL−1. Dedicated flow cytometry is capable of detecting single 102-nm polystyrene beads, as shown in Fig.1E. The width of the peaks is comparable to the reference bead PSD. Figure1F shows that the first bin of the vesicle standard PSD corresponds to 160nm. Consequently, dedicated flow cytometry detected approximately twice as small and thereby 18-fold more vesicles than conventional flow cytometry. The detected concentration was 3.3×108vesiclesmL−1. Figure1D,F was produced on the assumption of a vesicle refractive index of 1.40 14, 21, 22. Figure1G shows the reference bead PSD as detected by NTA. By combining two measurements with different settings, NTA detected all reference bead sizes, although only 5% of the 46-nm beads were detected. Tracking of 46-nm beads was hindered by the presence of larger beads that saturated the camera. Figure1G also shows that the peaks overlap because of broadening, which we attribute to the uncertainty in the measured diffusion coefficient, resulting from a limited track length and the uncertainty in the particle position. Figure1H shows the vesicle standard PSD obtained by combining two different settings. The peak at 95nm is broad as compared with other vesicle PSDs. The smallest detectable vesicles appear to be 10nm, which we attribute to broadening of the PSD. Using identical settings, we could detect only 5% of the 46-nm polystyrene beads, which have comparable light scattering to a 70–90-nm vesicle. Figure1I shows the reference bead PSD as detected by RPS. Through combination of measurements with an NP100 and NP400 pore, beads of 102nm and larger were detected. The peaks are broadened as compared with the reference bead PSD, which may be caused by particle aggregation, electronic noise, and a varying pore dimension during the measurement. Figure1J shows the vesicle standard PSD with a peak at 75nm. The PSDs of vesicles are fitted by a mathematical function to enable quantitative comparison. To select the most appropriate function, we fitted the vesicle standard PSD with six empirical functions that are frequently used to describe PSDs of particles in suspension 16, and performed goodness-of-fit tests (DataS1). The Gamma function, Weibull distribution and power-law function resulted in the best fits. Of these functions, we selected the power-law function, as it is least susceptible to minimum detectable vesicle size. The right panels of Fig.1 show PSDs of the vesicle standards fitted by the power-law function (dashed lines). The measured reference bead PSDs in Fig.1 were fitted by a sum of Gaussian functions (dotted lines) to derive the mean and standard deviation of the size and the concentration for each subpopulation of beads (Table1). The symbols in Fig.3A indicate the relative measurement error of the size as the percentage difference between the measurement and the manufacturer specification. Because TEM data were used as reference, its relative size error is set at 0%. The relative size error of the other methods was 9%. Dedicated flow cytometry had the lowest error in sizing beads, followed by RPS, conventional flow cytometry, and NTA. We attribute the low error of flow cytometry to the homogeneous refractive index of polystyrene and the strong relationship between size and scattering power (Fig.2). The error of RPS was limited because of specific measurement restrictions, as described in Materials and methods. We attribute the relatively large error of NTA to the uncertainty in the measured diffusion coefficient. Figure 3Open in figure viewerPowerPoint Relative error (symbols) and coefficient of variation (CV) (error bars) in determining the size (A) and concentration (B) of TEM, conventional flow cytometry, dedicated flow cytometry, nanoparticle tracking analysis (NTA) and resistive pulse sensing (RPS) for 46-nm beads (black), 102-nm beads (red), 203-nm beads (blue), 400-nm beads (green) and 596-nm beads (brown) from the reference mixture. Subpopulations that could not be detected are indicated by red crosses. The error bars in Fig.3A indicate the CV, which is the percentage ratio between the standard deviation and the mean size, and is thus a measure of the width of the peaks in Fig.1. Owing to the high resolution of TEM as compared with the measured standard deviation of the bead sizes, this standard deviation is a close approximation of the actual size of the beads. The lowest CVs were obtained by dedicated flow cytometry, followed by conventional flow cytometry, RPS, and NTA. Figure3B shows the relative measurement error in determining the concentration of subpopulations of reference beads. RPS was most accurate in determining the concentration of beads, followed by conventional flow cytometry, dedicated flow cytometry, and NTA. The error of RPS was limited because of specific measurement restrictions. With flow cytometry, the concentration was derived from the flow rate, which has an uncertainty of 10%. Dedicated flow cytometry underestimated the concentration of 102-nm beads, as these beads were close to the detection threshold. NTA was the least accurate method for determining the concentration of beads, possibly because of broadening of the PSD and crosstalk between 203-nm beads and 400-nm beads. The concentration of 46-nm beads was underestimated, as tracking of 46-nm beads was hindered by the presence of larger beads that saturated the camera. Figure4 shows the detected concentration of vesicles per technique. As compared with RPS and NTA, conventional flow cytometry underestimates the concentration of vesicles almost 300-fold, whereas the more sensitive dedicated flow cytometer underestimates the vesicle concentration 15-fold. With TEM, the detected concentration was affected by sample preparation losses. Figure 4Open in figure viewerPowerPoint Total detected concentration (logarithmic scale) of vesicles detected by transmission electron microscopy (TEM), conventional flow cytometry, dedicated flow cytometry, nanoparticle tracking analysis (NTA), and resistive pulse sensing (RPS). In this study, we compared the abilities of five commonly used methods to determine the PSD of vesicles in suspension. A reference mixture of polystyrene beads with known PSD (Fig.1A) and a vesicle standard from urine (Fig.1B) were measured by each method. In agreement with our theoretical review 9, each technique gives a different PSD for the same sample. By comparing the vesicle PSDs and combining these results with the knowledge obtained from reference beads, however, many differences are now explained. Throughout the Results section, we have discussed the requirements and assumptions involved in the measured PSDs. Table3 summarizes these requirements and assumptions, and also lists the minimum detectable vesicle size, the measurement times, and the capabilities to obtain functional information, such as fluorescence. In the next section, we will discuss our approach and the results in more detail. Table 3. Assessed capabilities of techniques for the detection of single vesicles in suspension Calibration with beads Spherical particle, n=1.40±0.02 Calibration with beads Spherical particle, n=1.40±0.02 NTA, nanoparticle tracking analysis; RPS, resistive pulse sensing; TEM, transmission electron microscopy. For each technique, the minimum detectable vesicle size, ability to measure the size and concentration and the requirements for this, ability to detect additional features, and measurement time are estimated. We derived the minimum detectable vesicle size of RPS from six measurements performed with different NP100 pores. d is the vesicle diameter, ΔD is the uncertainty in the diffusion coefficient, Iv and Ib are the scattering intensities of a vesicle and a calibration bead, respectively, η is the viscosity of the solvent, n is the vesicle refractive index, Q is the flow rate, QP is the flow rate caused by external pressure, σv are σm are the electrical conductivities of a vesicle and the medium, respectively, and T is the temperature of the solvent. The measurement time is indicated by S, M, and H, meaning 1min, between 1min and 1h, and 1h, respectively. To obtain the size of the reference beads (Fig.1A), we used traceable TEM measurements of the manufacturer. Traceability means that the measurement result is related to SI units through an unbroken chain of comparisons with known uncertainties 26, 27. To characterize beads, TEM is particularly useful, as beads are not affected by sample preparation, and the resolution of TEM is higher than the size of the beads. For comparison purposes, we set the relative size error of TEM to 0 (Fig.3A). However, the relative size error of the reference beads ranges from 1.0% for the 596-nm beads to 4.3% for the 46-nm beads. The TEM data also provide the CV of the beads, which is a measure of the spread in bead sizes. Consequently, the error bars in Fig.3A represent not only the imprecision of the instrument, i.e. the broadening of the reference bead PSD caused by the instrument, but also the CV of the reference beads. We derived the reference bead concentrations from the manufacturer-specified mass concentration, density and size of the beads. Note that the concentration of submicrometer beads is not traceable, as uncertainties in the mass concentration and density of the beads are unknown. The mass concentration is often provided with single-digit precision, and the density of silica beads may range from 1.8 to 2.5gcm−3. Consequently, the bead concentration and error thereof are unknown, and the relative concentration errors can only be mutually compared (Fig.3B). TEM analysis of vesicles involves two centrifugation steps and extensive sample preparation. To quantify the influence of these preanalytic variables on the obtained PSD (Fig.1B), we overlapped the power-law functions of TEM and RPS, which required horizontal and vertical stretching of the RPS data with factors of 0.88 and 0.21, respectively. Considering RPS to be the most reliable method for determining the PSD of vesicles, we hypothesize that vesicles shrink by 12%, owing to fixation and dehydration, and that 21% of the vesicles are recovered after centrifugation and binding to the formvar coating. To relate the measured light scattering to a particle size, we calibrated flow cytometers by using beads and Mie theory (Fig.2), assuming spherical particles of known refractive index. As beads meet these criteria, Mie theory can be used to determine their PSD (Fig.1C,E), resulting in dedicated flow cytometry being the most accurate in sizing beads (Fig.3A). However, the refractive index of vesicles is probably heterogeneous and not exactly known 28, thereby affecting the PSD of vesicles obtained by flow cytometry (Fig.1D,F). For example, under the assumption that the vesicle refractive index is 1.40±0.02, the minimum detectable vesicle sizes are 270–600nm for conventional flow cytometry and 150–190nm for dedicated flow cytometry (Table3). We attribute the high concentration of vesicles 340nm obtained by conventional flow cytometry relative to other techniques to background counts. An advantage of flow cytometry is knowledge of the analyzed sample volume, such that the particle concentration can be determined without calibration with beads. The PSD of beads determined by NTA shows extensive broadening as compared with the other techniques (Figs.1G and 3A). In addition, the determined concentration of vesicles requires careful interpretation. The manufacturer or user calibrates the instrument with beads to relate the mean number of scatterers in the field-of-view to the concentration 23. This calibration is valid for a vesicle size that scatters the same amount of light as the calibration beads. The concentration of smaller vesicles is underestimated, whereas the concentration of larger vesicles is overestimated. Moreover, the concentration of beads is not traceable. To obtain the minimum detectable vesicle size of 70–90nm, we related the scattering of 46-nm polystyrene beads, which were at the limit of detection, to the diameter of vesicles by using Mie theory. Software often applies unknown and undesired operations to the data. For example, Fig.5 shows the reference bead PSD detected by NTA with the low-sensitivity settings and processed by nta v2.3.5.16 (Nanosight). Analysis of the videos with this newer software results in a PSD (blue line) different from that in Fig.1G (blue line). The software generates a batch summary file, wherein a rolling average is applied to the raw data, resulting in a smoother but less correct representation of the data (gray line). Application of finite track length adjustment (FTLA) results in narrower peaks, a decreased accuracy of the determined mean diameters, and the presence of an additional peak at 445nm (brown line). As FTLA introduces artefacts, the application of FTLA to polydisperse samples is not recommended. Figure 5Open in figure viewerPowerPoint Particle size distribution (PSD) of the reference beads detected by nanoparticle tracking analysis (NTA) with low-sensitivity settings and processed by nta v2.3.5.16 software (Nanosight). The bin width is 10nm. The software provides raw data (blue line), a rolling average of the data (gray line), and data processed with the finite track length adjustment (FTLA) algorithm (brown line), resulting in different PSDs. The FTLA algorithm results in a peak (brown arrow) that is absent in the rolling average of the data. Accurate sizing of vesicles by RPS requires that the electrical conductivity of a particle is negligible as compared with the conductivity of the electrolyte 16, 29. As polystyrene beads, cells and intact vesicles meet this requirement 30, we believe that the detected vesicle size is representative for urinary vesicles (Fig.1J). However, our measurement restrictions made the RPS measurements impractical. The major concerns with RPS are pore clogging and pore stability. In the case of pore clogging, we reversed the pressure or temporarily applied a high pressure with a plunger. As the pores are stretchable, plunging may change the pore dimensions, as observed by a change in the baseline current. If the baseline current changed by 5%, we repeated the measurement and calibration, resulting in a measurement time of several hours. The concentration is obtained by calibration with beads 29, which is inaccurate, because the concentration of used beads is not traceable. As the flow rate is mainly determined by pressure across the pore, and not by electro-osmosis or electrophoresis, the differences between the zeta potentials of vesicles and calibration beads are negligible. Consequently, the accuracy in determining the vesicle concentration is expected to be comparable to that for the mixture of beads. A biomarker based on vesicle enumeration should determine the concentration of a specific vesicle type. For this determination, the technique must obtain biochemical information to identify specific vesicles, and the measurement time should not exceed several minutes. Furthermore, size accuracy and precision are important, e.g. to distinguish vesicles from platelets. Our findings demonstrate that any reported concentration needs to be accompanied by the minimum detectable vesicle size. For example, the shaded area in Fig.1J shows that a decrease in the minimum detectable vesicle size from 80nm to 60nm would result in a 2.4-fold increase in the obtained concentration. Therefore, we propose daily monitoring of the minimum detectable vesicle size, as day-to-day variation is expected for each instrument. Alternatively, a power-law fit may be applied to compare concentrations obtained with different minimum detectable vesicle sizes. An additional requirement for comparison of concentrations is traceable determination of both size and concentration, which is problematic for techniques that calibrate the concentration with untraceable beads. In conclusion, each technique gave a different PSD for the same vesicle sample. Differences between the detected vesicle concentrations are primarily caused by differences between the minimum detectable vesicle sizes. The minimum detectable vesicle sizes were 70–90nm for NTA, 70–100nm for RPS, 150–190nm for dedicated flow cytometry, and 270–600nm for conventional flow cytometry. TEM could detect the smallest vesicles present, albeit after adhesion on a surface. Dedicated flow cytometry was most accurate in determining the size of reference beads, but is expected to be less accurate on vesicles, owing to heterogeneity of the refractive index of vesicles. A reliable estimate of the vesicle refractive index is required to convert the optical scatter signal detected by flow cytometry to size. Nevertheless, dedicated flow cytometry is relatively fast and allows multiplex fluorescence detection, making it most applicable to clinical research. E. van der Pol, R. Nieuwland, and T. G. van Leeuwen conceived and designed the research. E. van der Pol and A. E. Grootemaat acquired the data. C. Gardiner, E. van der Pol, F. A. Coumans, R. Nieuwland, and T. G. van Leeuwen interpreted the data. E. van der Pol, F. A. Coumans, and R. Nieuwland wrote the manuscript. All authors reviewed and made critical revisions to the manuscript. The authors would like to acknowledge E. van der Pol, Wageningen University, the Netherlands, for statistical support. Part of this work was funded by the EMRP (European Metrology Research Programme) under the Joint Research Project HLT02 (www.metves.eu). The EMRP is jointly funded by the EMRP participating countries within the European Association of National Metrology Institutes and the European Union. jth12602-sup-0001-Supplementary_material.pdfapplication/PDF, 606.4 KB Data S1. Methods and mathematical function to fit the particle size distribution of vesicles. Please note: The publisher is not responsible for the content or functionality of any supporting information supplied by the authors. Any queries (other than missing content) should be directed to the corresponding author for the article. 1Conde-Vancells J, Rodriguez-Suarez E, Embade N, Gil D, Matthiesen R, Valle M, Elortza F, Lu SC, Mato JM, Falcon-Perez JM. Characterization and comprehensive proteome profiling of exosomes secreted by hepatocytes. J Proteome Res 2008; 7: 5157– 66. 2Ratajczak J, Wysoczynski M, Hayek F, Janowska-Wieczorek A, Ratajczak MZ. Membrane-derived microvesicles: important and underappreciated mediators of cell-to-cell communication. Leukemia 2006; 20: 1487– 95. 3Simons M, Raposo G. Exosomes – vesicular carriers for intercellular communication. Curr Opin Cell Biol 2009; 21: 575– 81. 4van der Pol E, Böing AN, Harrison P, Sturk A, Nieuwland R. Classification, functions and clinical relevance of extracellular vesicles. Pharmacol Rev 2012; 64: 676– 705. 5Berckmans RJ, Sturk A, Schaap MC, Nieuwland R. Cell-derived vesicles exposing coagulant tissue factor in saliva. Blood 2011; 117: 3172– 80. 6Manly DA, Wang JG, Glover SL, Kasthuri R, Liebman HA, Key NS, Mackman N. Increased microparticle tissue factor activity in cancer patients with venous thromboembolism. Thromb Res 2010; 125: 511– 12. 7Rautou PE, Leroyer AS, Ramkhelawon B, Devue C, Duflaut D, Vion AC, Nalbone G, Castier Y, Leseche G, Lehoux S, Tedgui A, Boulanger CM. Microparticles from human atherosclerotic plaques promote endothelial ICAM-1-dependent monocyte adhesion and transendothelial migration. Circ Res 2011; 108: 335– 43. 8EL Andaloussi S, Maeger I, Breakefield XO, Wood MJA. Extracellular vesicles: biology and emerging therapeutic opportunities. Nat Rev Drug Discovery 2013; 12: 348– 58. 9van der Pol E, Hoekstra AG, Sturk A, Otto C, van Leeuwen TG, Nieuwland R. Optical and non-optical methods for detection and characterization of microparticles and exosomes. J Thromb Haemost 2010; 8: 2596– 607. Wiley Online Library 10van der Pol E, Coumans F, Varga Z, Krumrey M, Nieuwland R. Innovation in detection of microparticles and exosomes. J Thromb Haemost 2013; 11: 36– 45. Wiley Online Library 11de Vrij J, Maas SL, van Nispen M, Sena-Esteves M, Limpens RW, Koster AJ, Leenstra S, Lamfers ML, Broekman ML. Quantification of nanosized extracellular membrane vesicles with scanning ion occlusion sensing. Nanomedicine 2013; 1– 16. 12Dragovic RA, Gardiner C, Brooks AS, Tannetta DS, Ferguson DJP, Hole P, Carr B, Redman CWG, Harris AL, Dobson PJ, Harrison P, Sargent IL. Sizing and phenotyping of cellular vesicles using nanoparticle tracking analysis. Nanomedicine 2011; 7: 780– 8. 13Yuana Y, Oosterkamp TH, Bahatyrova S, Ashcroft B, Garcia RP, Bertina RM, Osanto S. Atomic force microscopy: a novel approach to the detection of nanosized blood microparticles. J Thromb Haemost 2010; 8: 315– 23. Wiley Online Library 14Konokhova AI, Yurkin MA, Moskalensky AE, Chernyshev AV, Tsvetovskaya GA, Chikova ED, Maltsev VP. Light-scattering flow cytometry for identification and characterization of blood microparticles. J Biomed Opt 2012; 17: 0570061– 8. 15van der Pol E, van Gemert MJC, Sturk A, Nieuwland R, van Leeuwen TG. Single versus swarm detection of microparticles and exosomes by flow cytometry. J Thromb Haemost 2012; 10: 919– 30. Wiley Online Library 16Jonasz M, Fournier GR. Light Scattering by Particles in Water, 1st edn. London: Academic Press, 2007. 17Nieuwland R, van der Pol E, Gardiner C, Sturk A. Platelet-derived microparticles. In: AD Michelson, ed. Platelets, 3rd edn. San Diego, CA: Academic Press, 2012: 453– 67. 18Yuana Y, Bertina RM, Osanto S. Pre-analytical and analytical issues in the analysis of blood microparticles. Thromb Haemost 2011; 105: 396– 408. 19Bohren CF, Huffman DR. Absorption and Scattering of Light by Small Particles. New York, NY: Wiley, 1983. 20Mätzler C. MATLAB functions for Mie scattering and absorption. 2002–11. 2002. Institut für Angewandte Physik. 21van Manen HJ, Verkuijlen P, Wittendorp P, Subramaniam V, van den Berg TK, Roos D, Otto C. Refractive index sensing of green fluorescent proteins in living cells using fluorescence lifetime imaging microscopy. Biophys J 2008; 94: L67– 9. 22Beuthan J, Minet O, Helfmann J, Herrig M, Muller G. The spatial variation of the refractive index in biological cells. Phys Med Biol 1996; 41: 369– 82. 23Gardiner C, Ferreira YJ, Dragovic RA, Redman CWG, Sargent IL. Extracellular vesicle sizing and enumeration by nanoparticle tracking analysis. J Extracell Vesicles 2013; 2: 1– 11. Wiley Online Library 24Théry C, Amigorena S, Raposo G, Clayton A. Isolation and characterization of exosomes from cell culture supernatants and biological fluids. Curr Protoc Cell Biol 2006; 30: 3.22.1– 22.29. Wiley Online Library 25Jensen OA, Prause JU, Laursen H. Shrinkage in preparatory steps for SEM – a study on rabbit corneal endothelium. Albrecht Von Graefes Arch Klin Exp Ophthalmol 1981; 215: 233– 42. 26Meli F, Klein T, Buhr E, Frase CG, Gleber G, Krumrey M, Duta A, Duta S, Korpelainen V, Bellotti R, Picotto GB, Boyd RD, Cuenat A. Traceable size determination of nanoparticles, a comparison among European metrology institutes. Meas Sci Technol 2012; 23: 125005. 27Varga Z, Yuana Y, Grootemaat AE, van der Pol E, Gollwitzer C, Krumrey M, Nieuwland R. Towards traceable size determination of extracellular vesicles. J Extracell Vesicles 2014; 3: 1– 10. Wiley Online Library 28Issman L, Brenner B, Talmon Y, Aharon A. Cryogenic transmission electron microscopy nanostructural study of shed microparticles. PLoS One 2013; 8: e83680. 29Kachel V. Electrical resistance pulse sizing: Coulter sizing. In: RA Hoffman, ed. Flow Cytometry and Sorting, 2nd edn. New York, NY: Wiley-Liss, 1990: 45– 80. 30Montesinos E, Esteve I, Guerrero R. Comparison between direct methods for determination of microbial cell-volume – electron-microscopy and electronic particle sizing. Appl Environ Microbiol 1983; 45: 1651– 8. The full text of this article hosted at iucr.org is unavailable due to technical difficulties. Please check your email for instructions on resetting your password. If you do not receive an email within 10 minutes, your email address may not be registered, and you may need to create a new Wiley Online Library account. Can\'t sign in? Forgot your username? Enter your email address below and we will send you your username If the address matches an existing account you will receive an email with instructions to retrieve your username
本文链接: https://www.ebiomall.cn/b269-immunology/info-1417913149.html
免责声明 本文仅代表作者个人观点,与本网无关。其创作性以及文中陈述文字和内容未经本站证实,对本文以及其中全部或者部分内容、文字的真实性、完整性、及时性本站不做任何保证或承诺,请读者仅作参考,并请自行核实相关内容。
版权声明 未经蚂蚁淘授权不得转载、摘编或利用其他方式使用上述作品。已经经本网授权使用作品的,应该授权范围内使用,并注明“来源:蚂蚁淘”。违反上述声明者,本网将追究其相关法律责任。
相关文章
2015-04-16
2016-02-19
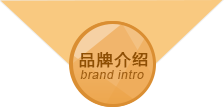
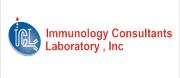
▍
品牌分类
▍
官网分类
▍
品牌问答