新闻动态
Organ-on-a-Chip - an overview | ScienceDirect Topics
2024-05-08
Skip to Main contentScienceDirectJournals BooksRegisterSign in Sign inRegisterJournals BooksHelpOrgan-on-a-ChipRelated terms:OrganoidProgenitor CellCell CultureMetabolismToxicityPharmacokineticsBioprintingEndothelial CellsNanoparticlesInduced Pluripotent Stem CellsView all TopicsDownload as PDFSet alertAbout this pageMicrofluidics in Cell Biology Part A: Microfluidics for Multicellular SystemsJong Hwan Sung, in Methods in Cell Biology, 2018AbstractOrgan-on-a-chip technology provides a novel in vitro platform with a possibility of reproducing physiological functions of in vivo tissue, more accurately than conventional cell-based model systems. Many newly arising diseases result from complex interaction between multiple organs. By realizing different organ functions on a chip, organ-on-a-chip technology is a potentially useful for building models of such complex diseases. Pharmacokinetic (PK) models provide a mathematical framework for understanding the interaction between organs involving transport and reaction of molecules. Here, we discuss various forms of organ-on-a-chip devices reported so far, with a particular emphasis on multi-organ devices for recapitulating multi-organ interactions. Also, we introduce the concept of PK models, and explain how it can be used to design and analyze multi-organ chip devices.View chapterPurchase bookRead full chapterURL: https://www.sciencedirect.com/science/article/pii/S0091679X18300591Regulation by non-coding RNAs in respiratory disordersAnkur Kulshreshtha, Anurag Agrawal, in Rna-Based Regulation in Human Health and Disease, 2020Organ-on-a-chip devicesOrgan-on-a-chip devices are microfluidic devices that are similar in principle to lung-organoids in having multiple cell-types, but they offer another layer of complexity by incorporating mechanical movements of the lungs. The repeated contraction and relaxation movement of lung to simulate physiological breathing motion is achieved using a cyclic vacuum suction to side chambers of cell-culture surface. This device consists of two channels, one is air channel and other is blood channel, offering a air-liquid interface similar to lung. This device was created using a microfabrication technique known as soft lithography [9].View chapterPurchase bookRead full chapterURL: https://www.sciencedirect.com/science/article/pii/B9780128171936000108Adult Stem Cells and Anticancer TherapyAudronė V. Kalvelytė, ... Aurimas Stulpinas, in Advances in Molecular Toxicology, 20174.2.3.5 Organs on chipsOrgan-on-a-chip systems in the nearest future may represent the most advanced miniaturized multiorganoid model that is expected to become an intermediate link between cell models and a human body. The principle of this technology is that multiple organoids of different cells may be organized together in a body-similar manner by the microfluidic connections. The main advantage of such systems is the integration of sensors that would allow monitoring physical, biochemical, and optical parameters in situ in real time. When the multisensor platform is developed, drug discovery, pharmacodynamic studies, and preclinical drug testing including patient-derived personal models will allow predicting the toxicity and the outcome of the treatment more accurately [350].3D cell culture models have proved to possess a number of undeniable advantages over 2D cell cultures. They have a great potential in fundamental research and in the pharmaceutical industry. Various studies show that the same cells cultured under 2D or 3D conditions differ in their morphological and physiological characteristics, leading to a different cellular response [177,178]. The ability of 3D cultures for intercellular and cellular matrix interactions affects signal transduction and gene expression. Consequently, cell behavior in 3D cultures is more similar to cellular response in vivo [179,206]. One of the main tasks in creating new models of a 3D cell culture is to ensure physiological relevance, high throughput, and reproducibility. Solving these problems will ensure good opportunities for a widespread industrial application of cell cultures and reduced use of laboratory animals during development of a new drug, including the management of drug toxicities toward normal stem cells.View chapterPurchase bookRead full chapterURL: https://www.sciencedirect.com/science/article/pii/B978012812522900004XHuman Stem Cells for Organs-on-ChipsIn Stem Cells (Second Edition), 201413.4 Human Organ-on-a-Chip Models for Certain DiseasesThe organ-on-a-chip approach is particularly relevant for diseases specific to humans for which no animal models are known. Organs such as the brain and the immune system are very typically human. For this reason, representative cell lines or animal model systems do not exist for many human neurological and psychiatric diseases, as well as for diseases in which the immune system plays a crucial role. These range from autoimmune diseases all the way to cancer. Just a few examples are amyotrophic lateral sclerosis, multiple sclerosis, depression, schizophrenia and psychosis, Alzheimer’s, diabetes, rheumatic diseases and diseases such as systemic lupus erythematosus (SLE), skin diseases, fibrotic diseases, and all types of cancer. However, in view of the very human nature of our immune system, which is involved in the evolution and progression of basically every disease, development of drugs for nearly all diseases may benefit from the organ-on-a-chip approach when based on stem cells from patients with the disease of interest, as will be discussed next.Will we be able to create models of depression or schizophrenia in this type of model? Time will tell, but both basic scientists and even funding agencies believe that it may be feasible, and the pharmaceutical industry is keen to see the outcome of this type of fundamental biomedical and bioengineering research.View chapterPurchase bookRead full chapterURL: https://www.sciencedirect.com/science/article/pii/B9780124115514000131PerspectiveJianghui Hou, in A Laboratory Guide to the Tight Junction, 20209.6.3 Bioprinting of organCurrent organ-on-a-chip models lack the geometry, complexity, and functionality important for recapitulating the organ physiology. Bioprinting, with which living cells can be precisely deposited together with hydrogel-base scaffolds, allows fabricating the 3D tissue structures resembling those in naturally developed organs (Ozbolat Yu, 2013). A bioprinted model of human vascularized proximal tubule consists of two adjacent conduits that are lined with confluent epithelium and endothelium, respectively, embedded in a permeable ECM and can be independently accessed using a closed-loop perfusion system (Fig. 9.6.4) (Lin et al., 2019). The bioprinted tissue exhibits active tubular-vascular exchange of solutes, for example, glucose and albumin, akin to native kidney proximal tubules. Because each bioprinted tubule can be independently perfused and recorded by external electrodes, the transport properties, including both transcellular and paracellular permeabilities, are interrogated on the single-tubule level, that is the gold standard for renal physiology, and was made possible only by the ex vivo tubule perfusion technique (Malnic, Klose, Giebisch, 1966).Figure 9.6.4. Bioprinted human proximal tubule. (A) Schematic view of the 3D bioprinting process. (B) Simple and complex 3D proximal tubules and blood vessels can be bioprinted. Bar: 10 mm. (C) Whole-mount immunofluorescence staining of the bioprinted tissue, in which Na+/K+ ATPase labels the proximal tubule, CD31 labels the blood vessel. Bar: 1 mm. The gap between the tubular and vascular conduits is ~70 μm. Inset: cross-sectional image of the two open lumens. Bars: 100 μm. (D) High-magnification images of the bioprinted tissue after staining. Bar: 100 μm. PT: proximal tubule; PTEC: proximal tubule epithelial cell; GMEC: glomerular microvascular endothelial cell.Reproduced with permission from Lin, N. Y. C., Homan, K. A., Robinson, S. S., Kolesky, D. B., Duarte, N., Moisan, A., amp; Lewis, J. A. (2019). Renal reabsorption in 3D vascularized proximal tubule models. Proceedings of the National Academy of Sciences of the United States of America, 116, 5399–5404.View chapterPurchase bookRead full chapterURL: https://www.sciencedirect.com/science/article/pii/B978012818647300009XA body-on-a-chip (BOC) system for studying gut-liver interactionJong Hwan Sung, in Methods in Cell Biology, 20204 Final remarksAlthough organ-on-a-chip, also known as microphysiological systems (MPS) are at a early developmental stage, their potential as novel in vitro model systems for drug screening and disease models have well been recognized (Skardal, Shupe, Atala, 2016). Having made notable successes in building models of individual organs, the next foreseeable direction is toward development of more complex multi-organ systems, where organ modules interact with one another mimicking the biological processes in the body (Lee Sung, 2018a, 2018b, Sung et al., 2019).Because the gut and the liver together play a central role in maintaining normal body functions, they have significant implications in the mechanism of many diseases. Building a physiologically realistic gut-liver model is expected to be an important step toward understanding diseases that have been difficult to overcome with current knowledge. Although the current gut-liver chip recapitulates only basic forms of gut-liver interaction, development of more realistic models of gut-liver system will provide a more complete picture of the gut-liver axis. To realize this goal, several remaining challenges need to be overcome, such as optimization of transport process between the gut and the liver, as well as in the tissues, and incorporation of other important components in the system, such as the vasculature and the immune system.View chapterPurchase bookRead full chapterURL: https://www.sciencedirect.com/science/article/pii/S0091679X20300030Microfluidics in Cell Biology Part A: Microfluidics for Multicellular SystemsJeonghwan Lee, ... Sejoong Kim, in Methods in Cell Biology, 20184.3 Organ Interaction and Disease ModelingMulti-organs-on-a-chip can be used to reveal systemic interactions between organs and investigate the treatment effects on multiple organs. Zhang and colleagues developed a compartmentalized 3D microfluidic cell-culture system containing liver, lung, and kidney cells and adipocytes (Zhang, Zhao, Abdul Rahim, van Noort, Yu, 2009). Four cell culture channels with each cell type were linked serially with common culture media flow. Investigators examined drug effects on each organ cell and evaluated inter-organ interactions using this microfluidic cell-culture system. Gelatin microspheres containing TGF-β1 were designed to regulate the release of TGF-β1. Culture media fluid was designed to flow through the lung cell compartment and the TGF-β1 microspheres into the other organ compartments. TGF-β1 activated the cells in the lung compartment without compromising the function of cells in the other organ compartments. This compartmentalized 3D microfluidic cell-culture system enabled the simulation of inter-organ connections, proved functional separation, and showed the possibility of utilization for potential application in human drug screening.Choucha-Snouber and colleagues designed a liver–kidney co-culture bio-chip and demonstrated the nephrotoxicity of hepatic metabolite of ifosfamide on kidney cells (Choucha-Snouber et al., 2013). Two culture chambers were connected to each other enabling culture medium in the hepatic chamber to flow into the kidney chamber. Hepatic (HepG2/C3a) and kidney (MDCK) cells were cultured in each chamber. In the liver–kidney bio-chip models, the number of MDCK cells decreased significantly due to the effect of the toxic metabolite of ifosfamide, chloroacetaldehyde, that was formed by the hepatic cells in the liver chamber. This two-organs-on-a-chip model showed the nephrotoxicity of a drug, proving the drug metabolite produced in liver as the cause of nephrotoxicity, and demonstrated the systemic organ–organ interactions.View chapterPurchase bookRead full chapterURL: https://www.sciencedirect.com/science/article/pii/S0091679X18300645Translational Pharmacology and Clinical TrialsMeina Li, Alastair G. Stewart, in Reference Module in Biomedical Sciences, 20212.2.2.3 Issues related to practical applications of body-on-a-chipIn addition to limitations we discuss above in currently established organ-on-a-chip devices (relatively low throughput, cell sources, biomaterial limitation and so on), there are still many other challenges in developing and establishing the utility of body-on-a-chip. The culture medium incompatibility issue between multiple organ chips is a major challenge. The experimental design is more difficult than single organ-on-a-chip experiment, as body-on-a-chip experiment design needs to ensure optimal organ-specific differentiation protocols that must be entrained over different time frames in parallel. Many other essential elements need to be taken into consideration for designing body-on-a-chip systems, such as developing instrumentation that can fluidically link and sustain the viability of different organs, developing physiologically relevant linking protocols to replicate fluid flow between different organs (Sontheimer-Phelps et al., 2019). There are also a variety of other practical and translational challenges including flow partitioning, flow rates, physiological sensors, sampling frequency and volumes. Therefore, despite the considerable advances made during the past decade, the aforementioned aspects remain to be improved before the full potential of these technologies can be realized.View chapterPurchase bookRead full chapterURL: https://www.sciencedirect.com/science/article/pii/B9780128204726000281Volume 1Aleksandra A. Golebiowska, ... Syam P. Nukavarapu, in Encyclopedia of Tissue Engineering and Regenerative Medicine, 2019Organ-on-a-ChipWith considerable advances in 3D cell culture systems and techniques, along with advancements in microfluidics, the \"organ-on-a-chip” systems have gained considerable notice. The ability to culture several cell types while mimicking in vivo conditions with the use of microfluidic channels have large implication in enhancing high throughput drug screening technologies (Huh et al., 2011). Also, the cell-to-cell interactions prevalent in these systems and precise control over microfluidics have led to the development of niche systems for a better understanding of how the cells function together (You et al., 2008). In context with bone tissue engineering, organ-on-a-chip systems have led to discoveries regarding bone tissue cells and their responses to mechanical and topological stimuli. The utilization of organ-on-a-chip system has been summarized below in Table 1. You et al. used an organ-on-a-chip system using oscillating fluid flow to simulate mechanical loading while culturing osteocytes and osteoclast precursors (Park et al., 2012). Through this, the authors were able to determine that osteocytes suppress osteoclast formation in response to mechanical loading through secretion of OPG (Osteoprotegerin, antagonist to osteoclast development). Microfluidic chambers were also used by Park et al. to compare the effects of mechanical stimuli on adipose and bone marrow-derived stem cells (Park et al., 2012). Each type of stem cells was loaded onto separate chambers and cultured under mechanical stimulation provided by pressurized air and flexible membrane over the culture (Amini et al., 2012b). When subjected to 1 psi for 10 min every 12 h, both adipose and bone marrow-derived stem cells showed increases in osteogenic markers, with bone marrow-derived stem cells outperforming the adipose-derived stem cells (Amini et al., 2012b). These microscale studies investigating cellular response can help advance bone tissue engineering by identifying key mechanisms involved in bone regeneration.Table 1. Organ-on-a-chip in bone tissue engineeringAuthorCell type/materialObservationsReferencesTissue modelingL. You et al.MLO-Y4 osteoclast-like cellsRAW264.7 monocyte osteoclast precursorsOsteocytes inhibited osteoclast differentiation when exposed to oscillating fluid flow through the OPG/RANKL pathwayYou et al. (2008)J. Lee et al.MC3T3-E1 preosteoblastsStaphylococcus epidermidisMicrofluidic channels development to determine efficacy of rifampicin eluting scaffolds on preventing bacterial infection. High-throughput method to evaluate infection preventionLee et al. (2012)S. Park et al.Human adipose-derived stem cells (hADSCs)Human bone marrow-derived mesenchymal stem cells (hMSCs)Mechanical stimulation of osteogenesis in both hADSCs and hMSCs. Higher sensitivity to mechanical stimuli in hMSCsPark et al. (2012)N. Jusoh et al.Human umbilical vein endothelial cells (HUVEC)Lung fibroblastsIncreased angiogenesis in response to increased hydroxyapatite and matrix stiffnessJusoh et al. (2015)Y. Hsu et al.Endothelial colony forming cell-derived endothelial cells (ECFC-ECs)Normal human lung fibroblasts (NHLFs)550, 620, and 775 mm in length channels to stimulate vascularization by seeded endothelial cells and fibroblasts to model human vasculatureHsu et al. (2013)Vascular tissue engineeringC. Fidkowski et al.Human umbilical vein endothelial cells (HUVEC)Poly(glycerol sebacate) (PGS)Capillary networks using PGS, a degradable polymer, ranging from 45–300 μm and cultured with HUVECS to observe endothelialization. Cells readily attached to the material and confluence along channel wallsFidkowski et al. (2005)J. Kim et al.Human endothelial progenitor cells (hEPCs)PLGA/Collagen I gelPLGA channels 1000 μm in width and 150 μm in height filled with Collagen I gel and loaded with hEPCs. After subcutaneous implantation, endothelial differentiation and vascularization was seenKim et al. (2014)A Golden and J. TienHuman dermal fibroblastsHuman dermal microvascular endothelial cells (HDMECs)Collagen I/gelatinCollagen I gel with microfluidic channels for vascular growth. Observed HDMEC cell attachment and proliferation on the channelsGolden and Tien (2007)Drug screeningSieber et al.Human hematopoietic stem and progenitor cells (HSPCs)Human mesenchymal stromal cells (MSCs)Hydroxyapatite coated zirconium oxide scaffoldHSPCs and MSCs co-cultured on hydroxyapatite coated scaffold within a microchannel device to simulate in vivo bone marrow conditions. The HSPCs demonstrated pluripotency and phenotypic maintenance after 28 days of in vitro cultureSieber et al. (2018))Torisawa et al.Hematopoietic cellsCollagen I gel with BMP-2 and 4Collagen I gel loaded with growth factors implanted subcutaneously to form a bone marrow-like system, where dense bone surrounded a blood-filled cavity. The cavity composed largely of hematopoietic cells survived without loss of \"stemness” for 1 weekTorisawa et al. (2014)Microfluidic channels have also shed much light in neovascularization, a key factor in bone tissue regeneration. Various studies developed scaffold structures and approaches for vascularized bone regeneration (Amini et al., 2012a; Tannoury and An, 2014; Amini and Nukavarapu, 2014; Hasan et al., 2014). Despite these efforts, scaffold-based bone tissue engineering strategies often lack proper angiogenesis to support large-area bone regeneration (Lovett et al., 2009; Sarker et al., 2015). In addition, scaffolds often develop a series of non-ordered channels that are insufficient in nutrient and waste transport to support regeneration (Sarker et al., 2015; Hsu et al., 2013). In response, microfluidic channels and micropatterning have shed much light on mechanisms and factors of neovascularization of bone tissues. Microfluidic channels that model human vasculature have been developed previously for modeling and drug screening purposes (Lovett et al., 2009; Sarker et al., 2015; Bhatia and Ingber, 2014; Jusoh et al., 2015). In particular, Hsu et al. have developed a model human vasculature ranging from 550, 620, and 775 mm in length composed of endothelial and stromal cells, and fibrin (Bhatia and Ingber, 2014). Vascularized tissue models for bone tissue in particular have also been developed using hydroxyapatite to model angiogenesis in context to hard bone tissue (Fidkowski et al., 2005). The chip consisted of endothelial cell channel and fibrin/hydroxyapatite channels separated but with junctions in between (Fidkowski et al., 2005). It was seen that the presence of hydroxyapatite promoted sprouting of endothelial cells into the fibrin matrix, and the observed angiogenesis is attributed to the stiffening of the fibrin matrix with hydroxyapatite addition (Fidkowski et al., 2005).In addition to mechanistic studies, these microfluidic techniques have also been utilized to create patterned scaffolds and degradable channels that can help guide vascularization in vivo (Lovett et al., 2009; Sarker et al., 2015). A variety of degradable polymers have been used to develop microfluidic channels conducive to vascularization, ranging from synthetic to natural biodegradable polymers, that may be incorporated into existing scaffold systems (Bettinger et al., 2006, 2007; Choi et al., 2007; Tang et al., 2004; Golden and Tien, 2007; Borenstein et al., 2010; Kim et al., 2014; Zhang et al., 2016; Collier et al., 2000). The microfluidic devices were developed using stereolithography to create a negative mold using PDMS or silicone, then casting with the degradable polymer, such as PLGA and PGS, to create the device (Sarker et al., 2015; Bettinger et al., 2006, 2007; Zhang et al., 2016). One strategy used PLGA to create the microfluidic channels which were then filled with collagen to aid endothelial cell seeding and vascularization (Zhang et al., 2016). It was seen that the microfluidic channels did show evidence of vasculature and capillary development when implanted in a subcutaneous mouse model for 4 weeks after 1 day of in vitro culture. Microfluidic channels have also been introduced to hydrogels using a sacrificial material to introduce the channels. Gelatin has been used as a sacrificial material to create microchannels in a collagen/fibrin gel with a diameter of ∼ 50 μm (Borenstein et al., 2010). The gelatin mold was made using stereolithography and PDMS negative mold, and the collagen/fibrin hydrogel was crosslinked around the gelatin, after which the gelatin was melted away at 37°C. The channels showed structural integrity and were able to support macromolecule transport as well as endothelial cell seeding. These techniques may provide beneficial when combined with existing osteogenic scaffold systems to also promote angiogenesis in the regenerated tissue.View chapterPurchase bookRead full chapterURL: https://www.sciencedirect.com/science/article/pii/B9780128012383110633The Key TechnologiesRobert D. Combes, in The History of Alternative Test Methods in Toxicology, 20192.3 Miniaturised and Large-Scale Culture SystemsMicrofabrication, micro-etching (soft lithography and hard micro-machining) and microfluidics have been adapted from the semi-conductor industry to generate organ-on-a-chip and organism-on-a-chip micro-scale culture systems, by using flexible, bio-compatible silicon and plastic wafer materials (5). Several chambers, each representing an organ, and containing mammalian cell cultures, interconnected by a fluid network, in microlitre, nanolitre and even picolitre volumes, can be constructed to permit intercellular communication and gaseous and nutrient exchange (6).Miniaturisation facilitates the replication of experiments under identical conditions, requiring small amounts of test chemical and media.At the other end of the size scale are large culture dynamic bioreactors comprising a 3-D network of interwoven hollow fibre membranes, which transport gases, nutrients, growth factors and by-products to and away from the cells, which can be in the form of mono-cultures or differentiated tissue constructs. Dynamic bioreactors show great promise for long-term toxicity testing (7).View chapterPurchase bookRead full chapterURL: https://www.sciencedirect.com/science/article/pii/B9780128136973000032Recommended publicationsInfo iconEngineeringJournalCell ReportsJournalAnimal Models for the Study of Human DiseaseBook • 2013CryobiologyJournalBrowse books and journalsAbout ScienceDirectRemote accessShopping cartAdvertiseContact and supportTerms and conditionsPrivacy policyWe use cookies to help provide and enhance our service and tailor content and ads. By continuing you agree to the use of cookies.Copyright © 2021 Elsevier B.V. or its licensors or contributors. ScienceDirect ® is a registered trademark of Elsevier B.V.ScienceDirect ® is a registered trademark of Elsevier B.V.
本文链接: https://www.ebiomall.cn/b427-progen/info-76749.html
免责声明 本文仅代表作者个人观点,与本网无关。其创作性以及文中陈述文字和内容未经本站证实,对本文以及其中全部或者部分内容、文字的真实性、完整性、及时性本站不做任何保证或承诺,请读者仅作参考,并请自行核实相关内容。
版权声明 未经蚂蚁淘授权不得转载、摘编或利用其他方式使用上述作品。已经经本网授权使用作品的,应该授权范围内使用,并注明“来源:蚂蚁淘”。违反上述声明者,本网将追究其相关法律责任。
相关文章
1970-01-02
2004-12-01
2009-03-24
2012-02-06
2012-08-08
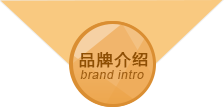
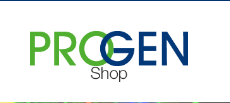
▍
品牌分类
暂无品牌分类
▍
官网分类
▍
品牌问答